Diversity and dynamics of clinical biofilms in ventricular assist device driveline infections and in vitro modelling
Yue Qu A B , David McGiffin C and Anton Y. Peleg A B D *A Infection Program, Monash Biomedicine Discovery Institute, Department of Microbiology, Monash University, Clayton, Vic. 3800, Australia.
B Department of Infectious Diseases, The Alfred Hospital and Central Clinical School, Monash University, Melbourne, Vic. 3004, Australia.
C Department of Cardiothoracic Surgery, The Alfred and Monash University, Melbourne, Vic. 3004, Australia.
D Centre to Impact AMR, Monash University, Clayton, Vic. 3800, Australia.
![]() Dr Yue Qu is a senior research fellow at the Department of Infectious Diseases, the Alfred Hospital and Monash University. His expertise is in translational research and contributed significantly to the field of medical device-related biofilm infections, from disease pathogenesis to prevention and treatment. His research has a broad coverage of bacterial and fungal pathogens, and different disease models, including bloodstream infections, vaginal candidiasis, VAD driveline infections, and many other medical device-related infections. |
![]() Prof. David McGiffin spent most of his career at the University of Alabama at Birmingham, where his major focus was thoracic transplantation, mechanical circulatory support and pulmonary endarterectomy. He returned to Australia in 2013 as Head of the Department of Cardiothoracic Surgery and Transplantation at the Alfred and Professor of Cardiothoracic Surgery at Monash University. He established a pulmonary endarterectomy program for chronic thromboembolic pulmonary hypertension at the Alfred, now the major referral program for Australia and New Zealand. |
![]() Prof. Anton Peleg FAAHMS is Director of the Department of Infectious Diseases at The Alfred Hospital and Monash University, Leader in the Centre to Impact Antimicrobial Resistance (AMR), Monash University, and Theme Leader for Infection and Immunity at the Monash Academic Health Research and Translational Centre. He completed his infectious diseases clinical training in Australia and then went to the USA and worked at the Harvard-affiliated hospitals, Beth Israel Deaconess Medical Center and Massachusetts General Hospital. He is a clinician–scientist with a research program that spans fundamental, translational and clinical research. |
Microbiology Australia 44(2) 83-87 https://doi.org/10.1071/MA23024
Submitted: 11 April 2023 Accepted: 4 May 2023 Published: 18 May 2023
© 2023 The Author(s) (or their employer(s)). Published by CSIRO Publishing on behalf of the ASM. This is an open access article distributed under the Creative Commons Attribution-NonCommercial-NoDerivatives 4.0 International License (CC BY-NC-ND)
Abstract
The important role of microbial biofilms in medical device-related infections is well established. Intervention strategies developed from in vitro biofilm studies often fail to prevent or cure device-related infections, possibly due to limited relevance of the simplified in vitro biofilm models to the much more complex clinical reality. It is important to use in vitro biofilm assays that closely mimic the dynamically changing clinical environment. This review uses ventricular assistant device driveline infections as a model of disease to demonstrate the morphological diversity and dynamics of clinical biofilms that are important for disease pathogenesis. We also provide insights into how to develop in vitro assays to address the complexity of device-related infections, focusing on pathogen-device interactions, infectious microenvironment, and selection of representative microorganisms and biomaterials.
Keywords: biofilm formation, biofilm migration, drip-flow reactor, driveline infections, infectious microenvironment, tunnel-based biofilms.
Introduction
Biofilms serve as a sophisticated infectious entity for many difficult-to-treat chronic infections, in particular those related to implantable medical devices. Formation of clinical biofilms is underpinned by host–device–pathogen interactions occurring at the specific anatomic sites of infection.1 Ventricular-assist device (VAD) driveline infections are a model of medical device-related infection with a known association with microbial biofilms.2 The driveline is a percutaneous tube connecting the pump and the extracorporeal controller unit of the VAD, conducting energy, controller algorithms and telemetric data. A typical HeartWare VAD (HVAD) driveline consists of an inner segment of smooth tubing made of polyurethane and another segment of outer tubing made of polystyrene velour (Fig. 1a, b), which is meant to enhance tissue integration of the embedded driveline. Driveline infections associated with VADs have a unique pathogenesis compared to other device-related infections such as catheter-related bloodstream infections or prosthetic joint infections. The exact mechanisms and factors involved in driveline infections are not fully understood, but recent research has started to uncover some important factors and interactions between the host, device and pathogen that contribute to the development and persistence of these infection.3–5 Unlike many other in vitro studies that focus on the genetic determinants of invading microorganisms,6,7 we studied pathogen–device interactions occurring around the implanted driveline and revealed ‘modifiable’ host, device and microbial factors that might determine the destiny of infection.3,4 It is now known that the diverse microenvironments around an implanted driveline, including the skin exit site, the driveline tissue tunnel and the velour–tissue interface, may facilitate the invading pathogens to form biofilms and migrate, with distinct characteristics along the driveline (Fig. 1a).3,4 It is challenging to mimic these biofilm infections in vitro. Here, we aim to illustrate the polymorphism of clinical biofilms in a single infection, using VAD drivelines as a model, and to shed light on how in vitro assays can be developed to closely mimic the dynamic in vivo environment.
(a) Ventricular assistant device (VAD), anatomic sites, biofilms and associated infections (reproduced with permission5). (b) An infected driveline explanted from a VAD patient.
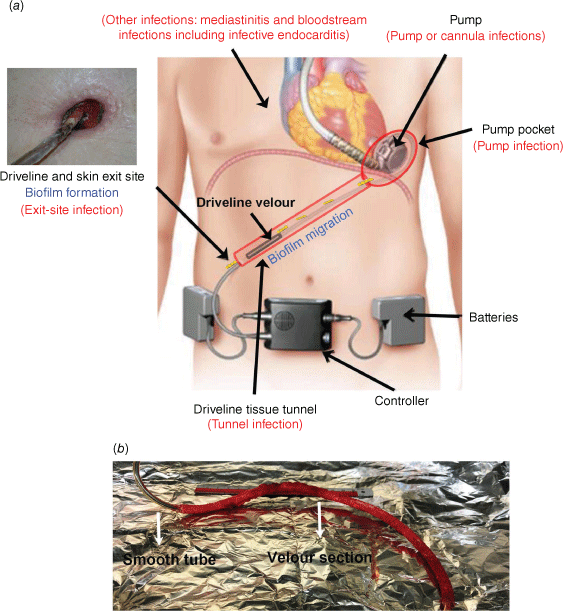
Drivelines support the formation of clinical and in vitro microbial biofilms
Clinical evidence that supports the importance of microbial biofilms in driveline infections was recently published.3,8 Using high-resolution scanning electron microscopy and quantitative colony-forming-unit enumeration assays, we examined explanted drivelines from patients with VADs who had driveline infections and control patients without infection. We found monolayer biofilms on the smooth tube section of the driveline at the skin exit site and microcolony biofilms on the velour at the tissue tunnel–driveline interface (Fig. 2). We also analysed in vitro biofilm formation of Staphylococcus aureus, Staphylococcus epidermidis, Pseudomonas aeruginosa and Candida albicans on the driveline materials under clinically relevant environmental conditions mimicking those encountered at the driveline skin exit site and in the driveline tissue tunnel and found the predilection of different pathogens to different parts of the driveline.4 The smooth tube of the driveline supported initial adherence of all four representative microbial species, with P. aeruginosa and C. albicans attaching to the tube surface at relatively higher densities. The scaffold provided by the three-dimensional structure of the driveline velour and the presence of the nutrient-rich driveline tunnel facilitated sporadically adhered S. aureus and S. epidermidis to form robust biofilms.4
Staphylococcal biofilm growths on an infected driveline at different anatomic sites, skin exit-site and driveline tissue tunnel (reproduced with permission3). Black arrows represent adherent monolayers and microcolony biofilms respectively.
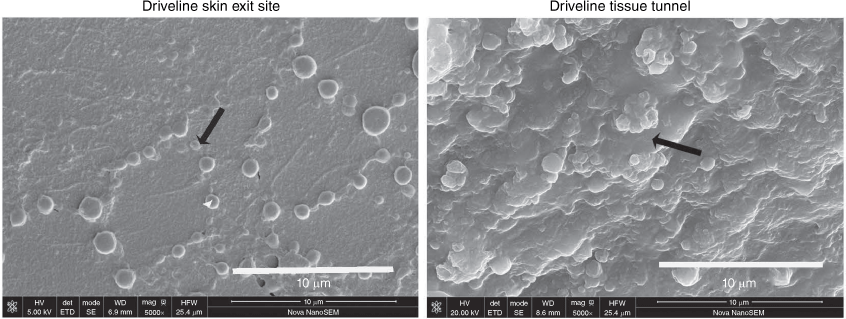
Biofilm formation at the driveline exit site and in vitro modelling
Driveline exit-site infection is the most common infection associated with VAD implantation and often presents as a chronic wound infection with a section of smooth tube or velour of driveline protruding from the skin exit site (Fig. 1a). Numerous in vitro and animal studies suggested that the formation of microbial biofilms at the skin exit site was the major cause of local driveline infections.4,6,7 The unique clinical environment of the driveline exit site is characterised by an open space for the device–pathogen interaction, adequate nutrient and oxygen supplies, and low-level shear stress. We speculated that the microbial interaction with the implanted driveline at the skin exit site was made up of two phases.4 Early adherence occurs soon after implantation, where microbial cells first encounter the surface of the driveline.9 To mimic this first phase, we developed an in vitro early adherence assay whereby microbial cells, resuspended in a drop of liquid media, interact statically with the driveline surface for 90 min in the presence of air (Fig. 3a). The second phase is the formation of mature biofilms on the device surface in an environment that mimics a chronic wound. Device–pathogen interactions during this phase occur at a solid–liquid–air interface. As wound drainage may provide a low shear force and continuous nutrient supply, an in vitro drip-flow biofilm reactor assay was adopted and further modified to mimic the exit-site wound environment, by reducing the liquid flow to a very low rate of 5 mL min−1 channel−1 (Fig. 3b).4,10 This in vitro assay allowed the growth of monolayer biofilms of S. aureus and S. epidermidis on the driveline materials, morphologically similar to that observed at the skin exit site of infected, explanted drivelines from patients (Fig. 2).3,4
Different biofilm assays mimicking diverse infectious microenvironment encountered in a driveline infection. (a) Early microbial adherence assay, (b) drip-flow biofilm reactor assay (reproduced with permission4), (c) tunnel-based biofilm growth assay and (d) tunnel-based biofilm migration assay. MHA, Mueller–Hinton agar; RPMI, Roswell Park Memorial Institute medium.
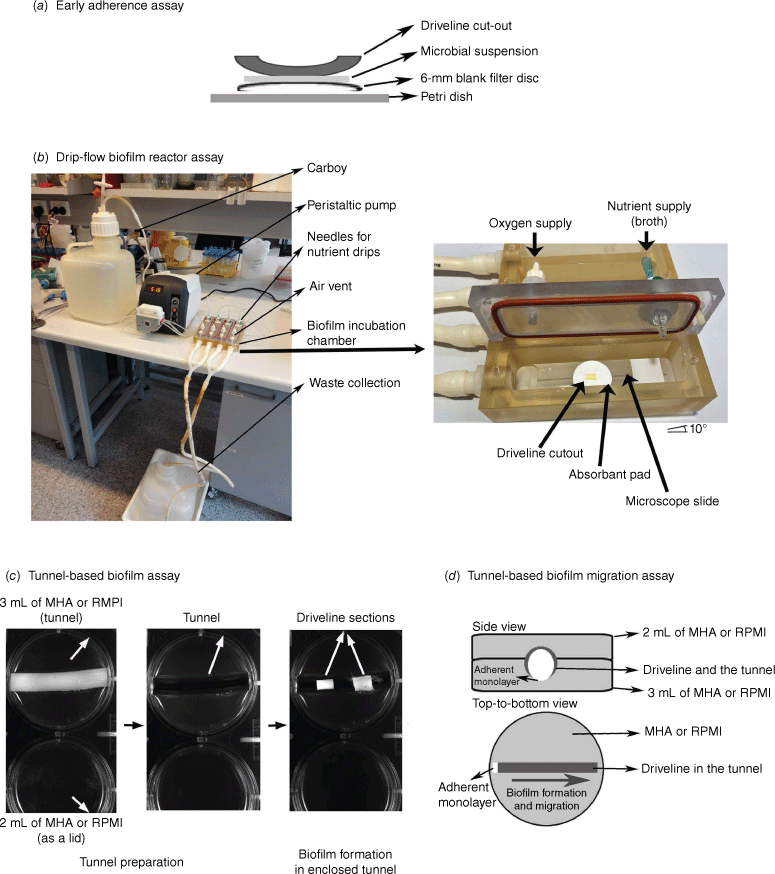
Formation of microcolony biofilms in the driveline tissue tunnel and in vitro modelling
Driveline tunnel infections are also frequently encountered.3,5,11 These infections are difficult to manage and often require surgical interventions such as debridement and re-tunnelling of the infected driveline.11,12 Examination of infected drivelines from patients with a VAD revealed microcolony biofilms of S. aureus and P. aeruginosa grown at the velour–tissue interface in the driveline tunnel.3 The driveline tunnel differs from the exit site in its confined space for biofilm growth, limited oxygen supply and rich nutrients from the surrounding tissues.4 Device–pathogen interactions in this environment occur at a solid–solid (tissue–device) interface with minimal liquid flow or shear force involved. We have developed a tunnel-based biofilm assay to closely mimic the tunnel environment (Fig. 3c). S. aureus and S. epidermidis that grow on drivelines as monolayer biofilms in the drip-flow biofilm reactor can form macro-colony biofilms in the enclosed agar tunnel, structurally resembling microbiology biofilms found on clinically infected drivelines explanted from the tissue tunnel.3
Biofilm migration and dynamics in driveline infections
The spread of driveline exit-site infection is known to cause more severe VAD-associated infections such as tunnel infection, bloodstream infection, pocket infection and pump infections.4,6,13 Our recent clinical study dissected infected drivelines from patients undergoing VAD explantation and heart transplantation, and revealed a microbial burden on driveline sections extending from the exit site to the deeper tissues, implicating biofilm migration along the driveline surface in the tissue tunnel.3 Two models of biofilm migration in the driveline tissue tunnel have been proposed, including the expansion of biofilms along the driveline surface and the releasing–seeding model. The latter allows the frontline of biofilms to release planktonic cells that further seed a remote area and regrow into a new biofilm. The expanding parental biofilms and newly grown biofilms eventually merge together and lead infections to the deeper tissues and parts of the driveline.
Host tissue integration of drivelines has been proposed as a defensive strategy against driveline infections.14 Driveline velour was designed by VAD manufacturers such as Abbott Medical and Medtronic to facilitate host tissue in-growth and to stabilise the driveline in the subcutaneous tunnel. In our analysis of explanted drivelines, we found numerous micro-gaps at the velour–tissue interface (incomplete tissue integration) in the driveline tunnel.3 It was hypothesised that these micro-gaps not only support the formation of a new biofilm at the driveline velour–tissue interface, but allow the biofilm to intermittently migrate to a remote area. Our newly published in vitro study has demonstrated the important role of micro-gaps contributing to biofilm formation and migration.15 Such micro-gaps may serve as a reservoir for oxygen and tissue fluid and support biofilm growth, and a transit station for biofilm migration. Our tunnel-based biofilm assay in combination with wide-field microscopy can be used to monitor real-time biofilm migration in a tissue tunnel4 (Fig. 3c, d). Micro-gaps can be fabricated in the agar tunnel using a surgical scalpel.15
Other important factors for in vitro modelling of complex biofilm infections
Representative biomaterials and microorganisms should be cautiously selected when modifying or developing in vitro biofilm assays to study a complex device-related infection. Tissue-culture treated polystyrene (TCPS) 96-well microplates have been widely used to study microbial biofilms, due to their commercial availability and low cost.16 However, they are likely far from ideal to study the complexity of a biofilm-related device infection. A standardised TCPS surface does not reflect the unique surface chemistry of biomaterials used to manufacture medical devices such as silicone- or polyurethane-based drivelines. The surface chemistry, in particular the elementary composition and the presence of specific functional groups, have been found to be a determinant for microbial biofilm formation on medical devices.17 For example, our recently published study found that the HVAD driveline made of Carbothane material was more resistant to biofilm formation by Staphylococcus spp. than its Pellethane predecessor.15 Furthermore, when designing in vitro experiments to mimic in vivo biofilm infections, the most common and representative microorganisms seen clinically should be selected. For in vitro modelling of driveline infections, staphylococcal species should be included as they have been reported to account for over 50% of driveline infections12,18 and other surgical site infections.19 Other clinically important, biofilm-producing microorganisms that should be considered are P. aeruginosa, C. albicans, Enterococcus faecalis, Klebsiella pneumonia and E. coli.16
Conclusion
This review uses VAD driveline infections as an exemplar of how to study pathogen–device interactions and biofilm formation and migration on implantable devices using in vitro assays that closely mimic dynamic infectious microenvironments in patients. Methodologies recommended by this review can be extended to other biofilm-related medical device infections, such as peritoneal dialysis catheter infections or pacemaker infections.
References
[1] Bjarnsholt, T et al. (2013) The in vivo biofilm. Trends Microbiol 21, 466–474.| The in vivo biofilm.Crossref | GoogleScholarGoogle Scholar |
[2] Salwiczek, M et al. (2014) Emerging rules for effective antimicrobial coatings. Trends Biotechnol 32, 82–90.
| Emerging rules for effective antimicrobial coatings.Crossref | GoogleScholarGoogle Scholar |
[3] Qu, Y et al. (2020) Characterization of infected, explanted ventricular assist device drivelines: the role of biofilms and microgaps in the driveline tunnel. J Heart Lung Transplant 39, 1289–1299.
| Characterization of infected, explanted ventricular assist device drivelines: the role of biofilms and microgaps in the driveline tunnel.Crossref | GoogleScholarGoogle Scholar |
[4] Qu, Y et al. (2020) Biofilm formation and migration on ventricular assist device drivelines. J Thorac Cardiovasc Surg 159, 491–502.e2.
| Biofilm formation and migration on ventricular assist device drivelines.Crossref | GoogleScholarGoogle Scholar |
[5] Qu, Y et al. (2021) Ventricular assist device-specific infections. J Clin Med 10, 453.
| Ventricular assist device-specific infections.Crossref | GoogleScholarGoogle Scholar |
[6] Toba, FA et al. (2011) Role of biofilm in Staphylococcus aureus and Staphylococcus epidermidis ventricular assist device driveline infections. J Thorac Cardiovasc Surg 141, 1259–1264.
| Role of biofilm in Staphylococcus aureus and Staphylococcus epidermidis ventricular assist device driveline infections.Crossref | GoogleScholarGoogle Scholar |
[7] Arrecubieta, C et al. (2009) SdrF, a Staphylococcus epidermidis surface protein, contributes to the initiation of ventricular assist device driveline-related infections. PLoS Pathog 5, e1000411.
| SdrF, a Staphylococcus epidermidis surface protein, contributes to the initiation of ventricular assist device driveline-related infections.Crossref | GoogleScholarGoogle Scholar |
[8] Schoenrath, F et al. (2021) Fluorescence in situ hybridization and polymerase chain reaction to detect infections in patients with left ventricular assist devices. ASAIO J 67, 536–545.
| Fluorescence in situ hybridization and polymerase chain reaction to detect infections in patients with left ventricular assist devices.Crossref | GoogleScholarGoogle Scholar |
[9] Qu, Y et al. (2010) Antibiotic susceptibility of coagulase-negative staphylococci isolated from very low birth weight babies: comprehensive comparisons of bacteria at different stages of biofilm formation. Ann Clin Microbiol Antimicrob 9, 16.
| Antibiotic susceptibility of coagulase-negative staphylococci isolated from very low birth weight babies: comprehensive comparisons of bacteria at different stages of biofilm formation.Crossref | GoogleScholarGoogle Scholar |
[10] Goeres, DM et al. (2009) A method for growing a biofilm under low shear at the air–liquid interface using the drip flow biofilm reactor. Nat Protoc 4, 783–788.
| A method for growing a biofilm under low shear at the air–liquid interface using the drip flow biofilm reactor.Crossref | GoogleScholarGoogle Scholar |
[11] Nienaber, JJC et al. (2013) Clinical manifestations and management of left ventricular assist device-associated infections. Clin Infect Dis 57, 1438–1448.
| Clinical manifestations and management of left ventricular assist device-associated infections.Crossref | GoogleScholarGoogle Scholar |
[12] Trachtenberg, BH et al. (2015) A review of infections in patients with left ventricular assist devices: prevention, diagnosis and management. Methodist Debakey Cardiovasc J 11, 28–32.
| A review of infections in patients with left ventricular assist devices: prevention, diagnosis and management.Crossref | GoogleScholarGoogle Scholar |
[13] Pinninti, M et al. (2014) Driveline insulation as a conduit for left ventricular assist device pocket infection. J Thorac Cardiovasc Surg 148, e135–e136.
| Driveline insulation as a conduit for left ventricular assist device pocket infection.Crossref | GoogleScholarGoogle Scholar |
[14] Pereda, D and Conte, JV (2011) Left ventricular assist device driveline infections. Cardiol Clin 29, 515–527.
| Left ventricular assist device driveline infections.Crossref | GoogleScholarGoogle Scholar |
[15] Qu, Y et al. (2023) Anti-infective characteristics of a new Carbothane ventricular assist device driveline. Biofilm 5, 100124.
| Anti-infective characteristics of a new Carbothane ventricular assist device driveline.Crossref | GoogleScholarGoogle Scholar |
[16] Qu, Y et al. (2017) Optimizing microplate biofilm assays to screen anti-infective surfaces. Trends Biotechnol 35, 3–5.
| Optimizing microplate biofilm assays to screen anti-infective surfaces.Crossref | GoogleScholarGoogle Scholar |
[17] Qu, Y et al. (2020) Hyperosmotic infusion and oxidized surfaces are essential for biofilm formation of Staphylococcus capitis from the neonatal intensive care unit. Front Microbiol 11, 920.
| Hyperosmotic infusion and oxidized surfaces are essential for biofilm formation of Staphylococcus capitis from the neonatal intensive care unit.Crossref | GoogleScholarGoogle Scholar |
[18] Bomholt, T et al. (2011) Driveline infections in patients supported with a HeartMate II: incidence, aetiology and outcome. Scand Cardiovasc J 45, 273–278.
| Driveline infections in patients supported with a HeartMate II: incidence, aetiology and outcome.Crossref | GoogleScholarGoogle Scholar |
[19] Seidelman, JL et al. (2023) Surgical site infection prevention: a review. JAMA 329, 244–252.
| Surgical site infection prevention: a review.Crossref | GoogleScholarGoogle Scholar |