Histomorphometric comparison of the gravid and non-gravid uterus at the time of birth in the tammar wallaby (Macropus eugenii): insights into the embryo-maternal interface
Marie K. Muenzenberger

A
B
C
Abstract
Tammar wallabies have a very short gestation which does not exceed the length of the estrus cycle. Direct contact between embryo and mother is established via a short-lived yolk sac placenta only in the last third of gestation. Therefore, an efficient embryo-maternal interface is required to ensure rapid embryonic growth. However, the morphology of the placenta at the time of birth is not well described in marsupials.
To study the morphology of the embryo-maternal interface, to compare the gravid and the non-gravid uterus at the time of birth and to examine the presence of polyploid trophoblast cells.
Histomorphometrical analysis of the uteri from light microscopic images. Quantification of the endometrial vascularization in samples stained with CD31 using AI machine learning. DNA content estimations of the giant trophoblast cell nuclei by Feulgen Image Analysis Densitometry.
In histological sections of the gravid endometrium, more tissue area was occupied by blood vessels than in the non-gravid endometrium, with subepithelial capillaries making up one-fourth of the vessel area in the gravid endometrium. The gravid uterus exhibited a 2.75-fold increase in surface area due to winding folds. Polyploidy of the giant trophoblast cells was confirmed. Giant trophoblast cells showed signs of degeneration.
The adaptations of the gravid uterus and the polyploidy of the trophoblast cells ensure sufficient embryo-maternal exchange. However, they seem to be self-limiting.
Morphology of the tammar wallaby trophoblast and endometrium prior birth is optimized for rapid embryonic growth during the brief interaction between maternal and fetal cells but the placenta seemingly cannot persist after the designated due time.
Keywords: endometrial gland, endometrium, marsupial, morphology, placenta, trophoblast, uterus, yolk sac.
Introduction
The tammar wallaby (Macropus eugenii) is a small species of macropodid marsupial that is often used for mammalian comparative research as its reproductive cycle, development, husbandry, and genetics are very well described (Tyndale-Biscoe and Renfree 1987; Renfree et al. 2011). The tammar wallaby, like all marsupials, has two completely separate uteri and cervices and three separate vaginas. Each uterus is under the endocrinological influence of its associated ovary. After mating, the embryo proceeds only to the blastocyst stage before entering embryonic diapause due to either the lactational suppression of the corpus luteum by the sucking stimulus of the newborn fetus in the pouch or by short day length at other times (Tyndale-Biscoe and Renfree 1987).
Abolition of the suckling stimulus during the breeding season can be artificially triggered by removal of the pouch young (RPY) thereby synchronizing pregnancies in experimental cohorts of animals (Gordon et al. 1988). After RPY the corpus luteum resumes its progesterone production and the dormant blastocyst continues its development within 3 days (Hinds and Tyndale-Biscoe 1982).
Only from day 18 RPY, the outermost shell coat, which encloses the embryo, dissolves (Denker and Tyndale-Biscoe 1986) and a non-invasive yolk sac placenta is established (Freyer et al. 2002). On day 26.5 RPY the female gives birth to a highly altricial young. With ovulation occurring on the contralateral ovary 25–40 h after birth (Renfree and Lewis 1996) the pregnancy length is thus shorter than the estrus cycle, which takes 30.6 days (Merchant 1979). Combined with the anatomical arrangement this allows the tammar wallaby to mate again within 24 h of birth.
Regardless of the brief pregnancy, the endometrium responds to the presence of the conceptus. The morphologic reactions of the uterus have been mostly studied during diapause and early pregnancy after reactivation of the embryo (Denker and Tyndale-Biscoe 1986; Freyer et al. 2002; Hickford et al. 2008; Laird et al. 2016, 2017). Until the time of shell coat dissolution, both uteri show a similar histomorphology (Denker and Tyndale-Biscoe 1986; Freyer et al. 2002; Laird et al. 2016, 2017). The high columnar cells of the luminal epithelium (Lamina epithelialis mucosae) (Denker and Tyndale-Biscoe 1986; Freyer et al. 2002; Laird et al. 2017) show microvilli and kinocilia (Freyer et al. 2002). In the underlying Lamina propria mucosae, underneath a subepithelial layer of densely packed stromal cells (Laird et al. 2017), a network of capillaries is located (Freyer et al. 2002). While there is a peak in abundance of uterine glandular cross-sections shortly prior diapause in both uteri (Laird et al. 2016), at the time of birth they are more abundant in the gravid than in the non-gravid endometrium (Laird et al. 2016). With implantation the luminal epithelial cells flatten (Denker and Tyndale-Biscoe 1986; Laird et al. 2016, 2017) and maternal capillaries increase in number and diameter and become fenestrated. They come to lie directly beneath the basal lamina of the luminal epithelium (Freyer et al. 2002).
The uterine epithelium and the trophoblast cells lie closely apposed forming a yolk sac placenta (Denker and Tyndale-Biscoe 1986). The embryonic part of the yolk sac placenta comprises of a bilaminar (BOM) and a trilaminar omphalopleure (TOM). The bilaminar part consists of an outer layer of trophoblast cells and an inner lining of endoderm cells, whereas the trilaminar part includes an additional layer of mesenchyme with embryonic vessels (Renfree 1973).
Considerably enlarged trophoblast cells with big nuclei are described in the BOM at the time of implantation (Denker and Tyndale-Biscoe 1986; Jones et al. 2014). It has been proposed that the nuclei of these giant cells might be polyploid (Jones et al. 2014). Occasionally, binuclear cells are found in the BOM trophoblast as well as in the endoderm (Jones et al. 2014).
Less information is available on the histological architecture of the non-gravid and gravid endometrium around parturition. Therefore, this study focuses on the description and comparison of the histomorphology of the gravid and the non-gravid uterus at the time of birth with emphasis on the quantification of endometrial structures. Thereby, we are not only interested in the maternal side but also the embryonic part investigating how the placenta enables rapid embryonic development. What are the morphological adaptations that ensure highly efficient nutrient and gas transfer, and what are their limitations?
Materials
Animals
The samples of tammar wallaby uteri examined in this study were collected in the course of another study (Drews et al. 2013; Buentjen et al. 2015). All experimentation was approved by the University of Melbourne Institutional Animal Ethics Committees and conformed to the National Health and Medical Research Council of Australia (2004) guidelines.
In this other study, the experimental group was treated with indomethacin to prevent birth as described previously (Renfree et al. 1994). As consequence, fetal death was detected by ultrasonography after a mean pregnancy length of 26.3 days (n = 8). Females of the experimental group were euthanized by i.v. injection of 60 mg pentobarbitone (60 mg/mL; 3–4 mL/kg) in the lateral tail vein. For easier differentiation, the embryo-bearing uterus will be referred to as ‘gravid’ and the contralateral ‘empty’ uterus will be addressed as ‘non-gravid’. The reproductive tract was removed, and strips of uterine tissue were collected. For one animal only embryonic tissues have been obtained. In total, four non-gravid uteri and four gravid uteri samples were included in this study (Table 1).
Animal number | Collected tissues | ||||
---|---|---|---|---|---|
Gravid uterus | Non-gravid uterus | BOM | TOM | ||
1 | x | – | – | x | |
2 | – | x | – | – | |
3 | x | – | x | x | |
4 | x | – | x | x | |
5 | – | x | – | – | |
6 | x | x | – | x | |
7 | – | x | – | – | |
8 | – | – | – | – |
BOM, bilaminar omphalopleure; TOM, trilaminar omphalopleure.
Tissue preparation and hematoxylin-eosin staining
After collection, samples were immediately placed in 4% paraformaldehyde in phosphate-buffered saline (PBS) for 12 h at 4°C and subsequently rinsed with PBS. Samples were then dehydrated in 70% ethanol with two replacements after 2 h. Dehydration proceeded in further ascending alcohol series before the tissues were embedded in paraffin. Samples were sectioned into 2 μm sections and placed on color-frosted microscope slides (Jiangsu Huida Medical Instruments Co., Ltd, Yancheng, China) for the hematoxylin-eosin stain (HE) and on SuperfrostTMPlus adhesion microscope slides (Epredia Netherlands B.V., DA Breda, Netherlands) for all other staining procedures.
Sections were deparaffinized and rehydrated using Neo-Clear™ (Merck KGaA, Darmstadt, Germany) or UltraClear™ (Avantor Performance Materials Poland SA., Gliwice, Poland) as intermedium and decreasing ethanol concentrations. HE slides were stained with filtered Haemalaun Meyer and Phloxin/Eosin solution and mounted with Neo-Mount™ (Merck KGaA, Darmstadt, Germany) or CVUltra (Leica Biosystems Richmon Inc, Richmond, USA), respectively.
Feulgen staining
For the Feulgen stain, after deparaffinizing and rehydrating, the slides were transferred via demineralized water into a 5 M HCl solution, where they were incubated for 50 min. Afterwards they were washed twice for 2 min in demineralized water and incubated for another 60 min in Schiff’s reagent (Morphisto GmbH, Offenbach, Germany). A special rinsing solution was prepared containing 5 mL of 10% sodium bisulfite and 1 mL 5 M HCl in 95 mL demineralized water. With this rinsing solution the slides were washed twice for three minutes each, before being transferred into pure demineralized water for another two times two minutes. After dehydration, the sections were mounted with Neo-Mount™ (Merck KGaA, Darmstadt, Germany).
Immunohistochemistry
An immunohistochemistry was performed using a polyclonal rabbit anti-human CD31 primary antibody (NB 100-2284, Novus Biologicals USA, Centennial, USA) to label endothelial cells. Antigen-retrieval was performed in citrate buffer 10 mM at pH 6 (C6) in a PELCO BioWave® Pro microwave (Ted Pella, Redding, Canada) after deparaffinization and rehydration. Buffer on its own was preheated to 95°C with 750 W for 2 min. For antigen retrieval, samples were then heated to 95°C at 750 W for 8 min, followed by another 10-min microwaving step at 550 W to maintain the samples at 80°C. Before further processing, the slides were left to rest for 20 min outside the microwave in the still hot C6 buffer to cool down to room temperature. A Novolink Polymer Detection System kit with goat anti-rabbit secondary antibody and diaminobenzidin (Leica Biosystems Newcastle Ltd, Newcastle upon Tyne, United Kingdom) was used according to the instruction protocol provided by the company. The primary antibody was diluted 1:100 in antibody diluent (9 mL demineralized water, 1 mL tris-buffered saline, 100 μL NaN3, 50 mg sodium caseinate) with 5% normal goat serum, and sections were left to incubate overnight at 4°C.
Positive and negative controls were included to identify any non-specific staining. Rat liver samples from the archival tissue bank of the Division of Veterinary Anatomy (Vetsuisse faculty, University of Bern, Bern, Switzerland) were used as positive controls. For the negative control, instead of the diluted primary antibody, only the antibody diluent was applied to rat liver and wallaby uterus samples, respectively.
Morphometric analysis
Sections were documented with a Zeiss Axio Imager with Apotome microscope (Carl Zeiss AG, Feldbach, Switzerland). All measurements were performed using the NIS-elements AR software (Nikon, Shinjuku, Tokyo, Japan).
In each sample, the thickness of the endometrium and the myometrium was measured in 20× magnification in three different HE slides and at three different locations per slide. Only sections showing the three uterine layers (endometrium, myometrium and perimetrium) in completion were used for the measurements. Between the two roughly parallel surfaces of each layer, a perpendicular transect was measured as described before by Cruz and Selwood (1997). Likewise, following earlier procedures by Laird et al. (2016) the uterine glandular profile was determined by counting all cross-sections of glands in counting frames of 0.52 × 0.52 mm. Cross-sections touching the edges of the frame were included. For each uterus sample three slides with three frames each were evaluated.
To quantify the endometrial vascularization and the proportion of the subepithelial capillaries within, three slides per sample in 40× magnification stained with CD31 were examined. To define a comparable endometrial area in each slide, a reference line with a length of 500 μm was drawn as parallel as possible to the endometrial-myometrial interface. From both ends of the reference line orthogonal side, lines up to the luminal epithelium were drawn. The length of the endometrial-myometrial interface and the luminal epithelial interface were indicated by connecting these two side lines, following closely the borderline between the myometrium and the endometrium and the apical margin of the luminal epithelium (Lamina epithelialis mucosae), respectively. The intersection of the sidelines with the endometrial-myometrial interface line and the luminal epithelial line confined our region of interest (ROI) (Fig. 1). This ROI area represented the evaluated endometrial tissue area.
Quantification of endometrial vascularization in light micrographs of anti-CD31 immunohistochemistry. The region of interest (ROI) is confined by the luminal epithelial surface (magenta), the endometrial-myometrial interface (yellow) and the side lines (dark green) perpendicular to the reference line (light green) connecting the ends of the endometrial-myometrial interface. The uterine glands (cyan) have been manually segmented. Blood vessel (red) segmentation was performed by the Artificial Intelligence software NIS-elements AR (Nikon, Shinjuku, Tokyo, Japan). Non-gravid (a), gravid (b), gravid non-segmented (c).
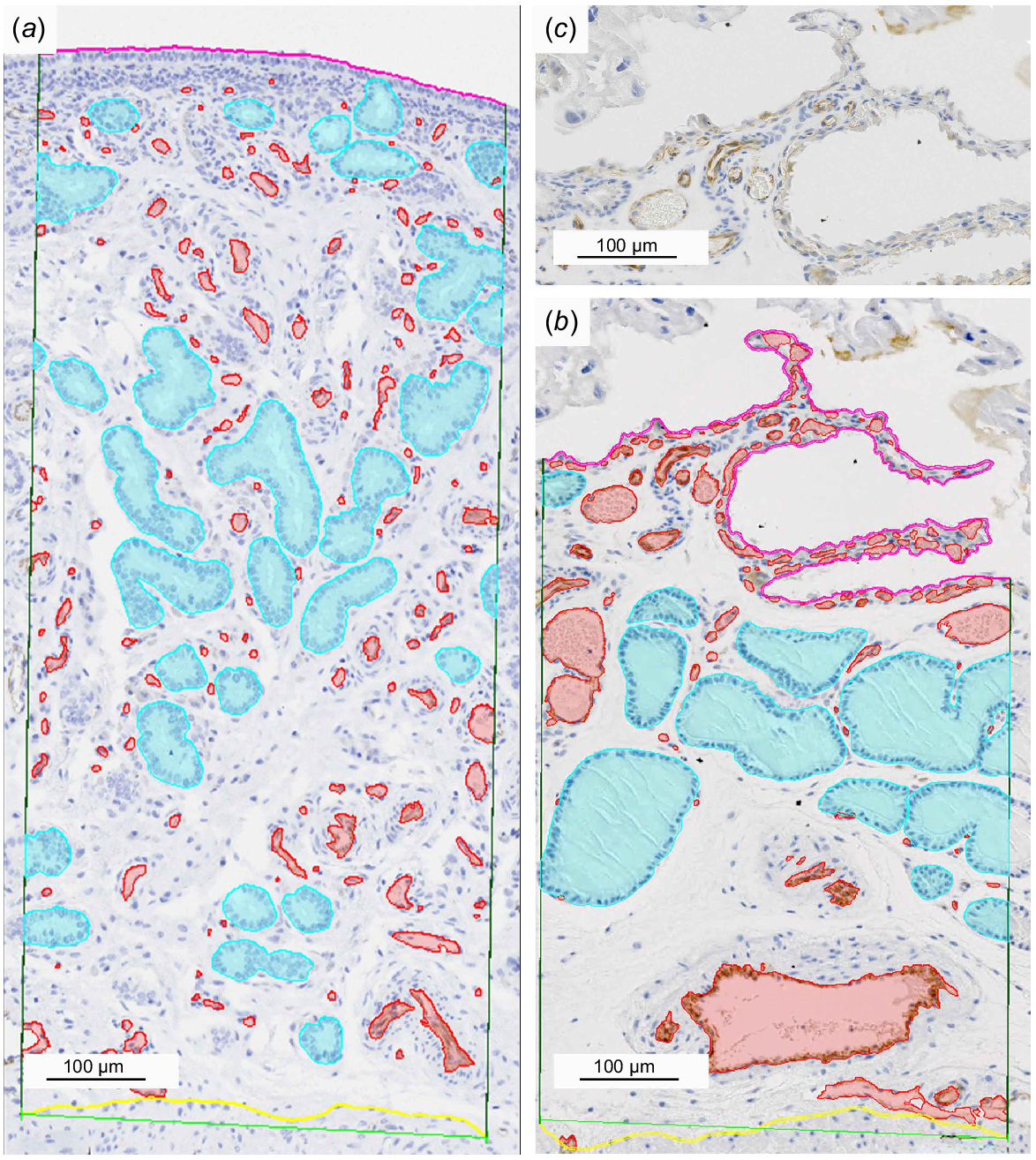
To calculate the total blood vessel area within the ROI the artificial intelligence (AI) software, which is part of the NIS-elements AR software (Nikon, Shinjuku, Tokyo, Japan), was trained via machine learning to automatically segment the blood vessels based on the CD31-labelled endothelial cells. To test the quality of the AI-segmentation, eight images (one for each sample), which were not included in the training, were segmented manually and by the AI and compared, showing an accuracy of 98%. The total blood vessel area in relation to the total endometrial tissue area within the ROI’s was calculated for each sample.
To determine the proportion of the subepithelial capillaries in the total evaluated blood vessel area another line parallel to the basal poles of the luminal epithelium in a distance of 15 μm was drawn indicating the basal boundary of the subepithelial layer. The thereby enclosed capillaries were then segmented by the AI and the subepithelial capillary area was measured.
Finally, all sections of the uterine glands enclosed in the ROI were segmented manually and the gland area was calculated. This was set in relation to the endometrial tissue area of the ROI and the correlation between the endometrial blood vessel area and area of uterine gland sections was evaluated.
To investigate if there is an increase in the epithelial surface area due to endometrial fold formation in the gravid uterus compared to the non-gravid uterus, an interface ratio between the luminal surface and the endometrial-myometrial interface was calculated using the following formula:
Evaluating polyploidy levels using Feulgen image analysis densitometry
For the following calculations it is necessary to include nuclei with a known DNA content to which the results of the unknown wallaby tissue can be compared. Horse skin tissue from the archival tissue bank of the Division of Veterinary Anatomy (Vetsuisse faculty, University of Bern, Bern, Switzerland) with a known genome size derived from the genome size database (Gregory 2024) was used as a standard and included in the staining procedure.
The following procedure was executed based on the instructions provided by Hardie et al. (2002).
As we performed the Feulgen image densitometry on histological sections (Zybina et al. 2009) we never evaluated the whole nucleus but only parts of it. Therefore, we can only estimate polyploidy levels. Although this needs to be acknowledged, it is still a valid approach as we used the same method for all three of our cell types, namely the horse glandular epithelial cells, the tammar wallaby glandular epithelial cell nuclei and the BOM trophoblast giant cell nuclei, and set them in relation to each other.
Sections were viewed and photographed with a Zeiss Axio Imager with Apotome microscope (Carl Zeiss AG, Feldbach, Switzerland) in 100× magnification with oil immersion.
Genome size estimations were executed using 60 BOM trophoblast giant cell nuclei and 60 uterine glandular epithelial cell nuclei of two animals (Table 1) in six sections. Pixel intensity values were provided by the NIS-elements AR software (Nikon, Shinjuku, Tokyo, Japan), all further calculations were performed in R Studio (R Core Team 2022).
The integrated optical density (IOD) was calculated for each nucleus separately as suitable nuclei lay too far apart to include several into one field for calculation. Nuclei were appointed ‘suitable’ if they were neither damaged nor overlapping with other nuclei and presented as completely as possible. The following formula was used:
with n being the number of pixels in the nucleus, IFi being the intensity of pixels within the nucleus and IBi being the intensity of background pixels. Pixel intensity was measured in green-cha. For IBi, a mean of the pixel intensities of a standardized ROI of an area without any tissue was inserted, always taken from the same image as the corresponding IFi values.
The above calculation was performed for the wallaby BOM trophoblast giant cell nuclei, the wallaby uterine glandular epithelial cell nuclei and the horse glandular epithelial cell nuclei, respectively.
Each set of IODs was separately sorted and, according to the instructions, the top and bottom 5% excluded to minimize the risk of outliers altering the final result before calculating a mean IOD. In the next step, the DNA content (CV) for the two wallaby cell types (u – unknown, s – standard) were estimated based on the known DNA content of the horse glandular epithelial cell nuclei:
The standard IOD, IODs, indicated the mean IOD of the horse uterine glandular epithelial cell nuclei and the unknown IOD, IODu, was either the IOD of wallaby glandular epithelial cells or wallaby trophoblast giant cells. For the determination of the CVu, for the CVs the known DNA content of the standard was inserted. This calculation was executed for every IOD of the trophoblast giant cells individually and a mean DNA content was calculated from the separate values. In the genome size database, there are several different estimates for the horse genome size. We decided to use a mean of these values for our calculations (1C = 3.19 pg). Furthermore, since we presumed the trophoblast giant cell nuclei to be polyploid, we calculated with the actual genome size of the diploid horse cell (2C = 6.38 pg). The BOM trophoblast value was expected to be a multiple of the value for the wallaby uterine glandular epithelial cells.
Statistical analysis
All statistical analyses were performed in NCSS 2024 Statistical Software (2024). The following hypotheses were tested regarding our measurements:
There is a significant difference in endometrial thickness between the gravid and non-gravid uterus.
There is a significant difference in myometrial thickness between the gravid and non-gravid uterus.
There is a significant difference in the number of uterine gland sections in the gravid and the non-gravid uterus.
There is a significant difference in the endometrial vessel area in relation to the endometrial tissue area in the gravid and the non-gravid uterus.
The uterine gland area correlates to the endometrial blood vessel area.
The surface of the luminal epithelium is greater in the gravid than the non-gravid uterus.
There is a significant difference in the subepithelial capillary area in relation to the total endometrial vessel area in the gravid and the non-gravid uterus.
To test for normality, the Shapiro–Wilk test was performed. At α ≤ 0.05 normality was rejected. Depending on normality, an independent sample t-test (normally distributed) or a two-sample Wilcoxon (Rank sum) test (not normally distributed) was performed for the hypotheses one to four, six, and seven. If the reported P-value was less than 0.05, the difference was considered to be statistically significant. For hypothesis five, a Pearson correlation coefficient (r) was calculated where r = −1 indicates a perfect negative correlation and r = 1 indicates a perfect positive correlation.
Results
Morphology of the non-gravid uterus
The endometrium composed of the luminal epithelium (Lamina epithelialis mucosae) and the underlying layer Lamina propria mucosae showed a mostly flat surface and exhibited a total height of 1.26 mm (s.e.m. = 0.06).
The Lamina epithelialis mucosae comprised of a single layer of cubic to columnar cells bearing protrusions. Some protrusions of the ciliated cells appeared to be either kinocilia or stereocilia due to their apparent lower frequency and greater length compared to microvilli. (Fig. 2b). Numerous intraepithelial lymphocytes were present (Figs 2c, 3a).
Light micrographs of the non-gravid uterus at the time of birth stained with hematoxylin and eosin. Overview (a), focus on the luminal epithelium and subepithelial area (b, c). bc, brown macrophage-like cell; c, subepithelial capillary; em, endometrium; gl, uterine gland; IEL, intraepithelial lymphocyte; k, kinocilia; le, luminal epithelium; mm, myometrium; pm, perimetrium; smc, Stratum musculare circulare; sml, Stratum musculare longitudinal; v, vessel; green star, dense cell layer.
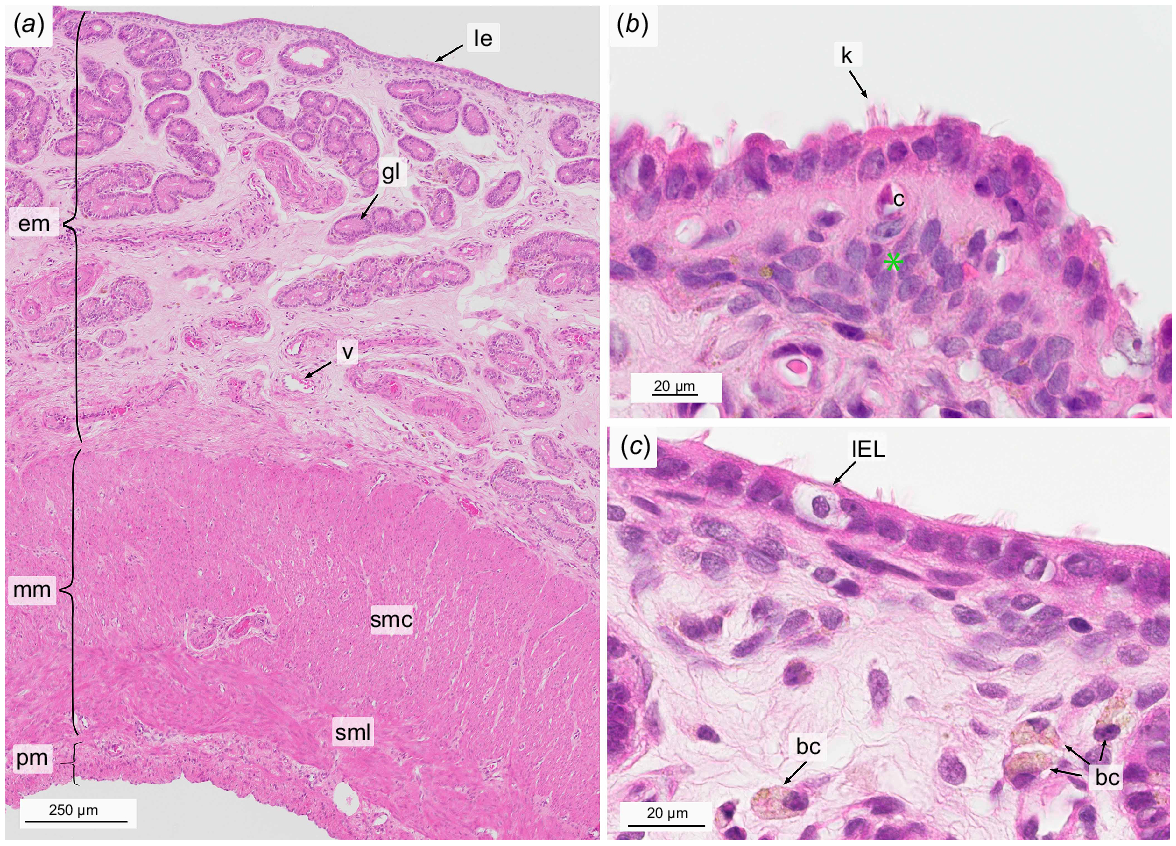
Light micrographs of intraepithelial lymphocytes (a) and superficial uterine glands (a, b) in the non-gravid uterus stained with hematoxylin and eosin. gl, uterine gland with protrusions; IEL, intraepithelial lymphocyte.
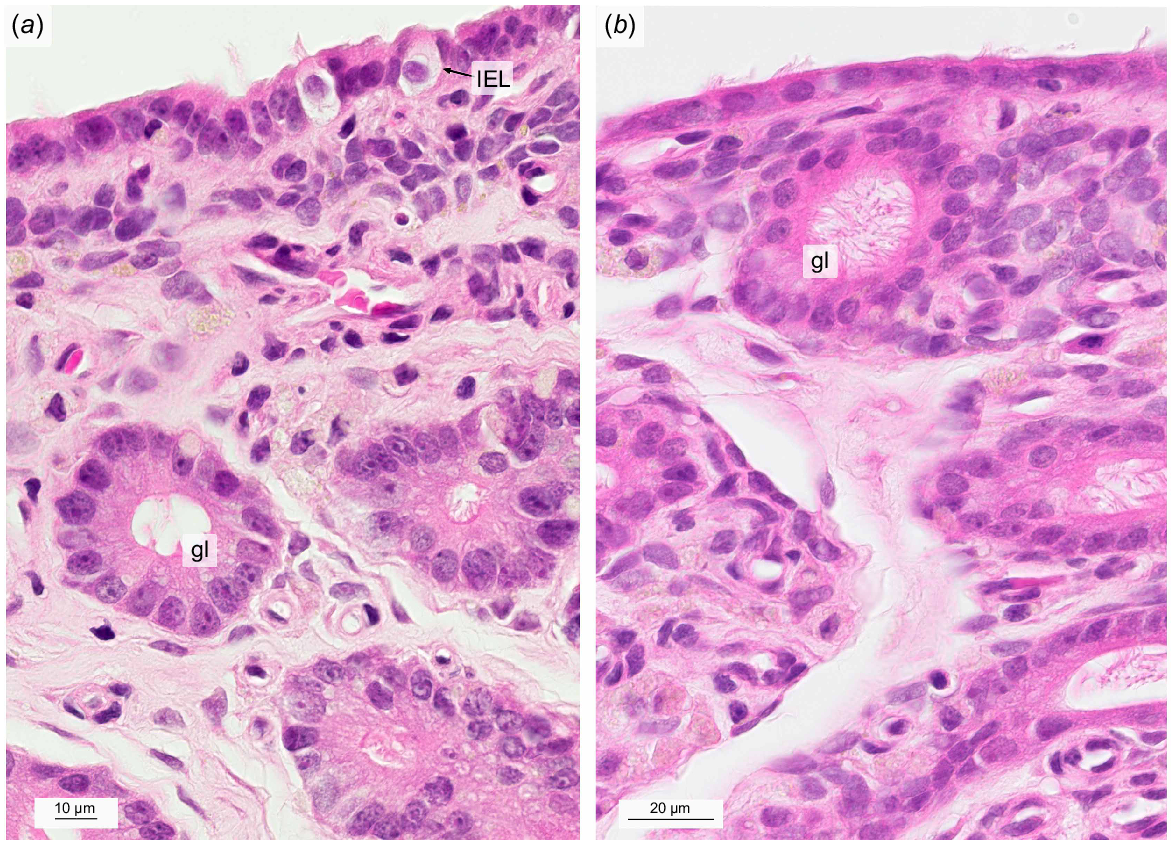
The underlying Lamina propria mucosae could be divided into three layers: a compact, cell-rich subepithelial layer, a middle layer of loose connective tissue, and a layer towards the myometrium where the connective tissue was dense and contained numerous large blood vessels (Fig. 2a). Within the compact subepithelial layer a small number of subepithelial capillaries was located (Fig. 2b), which made up 1.13% (s.e.m. = 0.29) of the ROI endometrial blood vessel area. The latter covered 3.92% (s.e.m. = 0.51) of the quantified endometrial area (Table 2).
Non-gravid | Gravid | Statistical significance | ||||
---|---|---|---|---|---|---|
Mean | s.e.m. | Mean | s.e.m. | P-value A | ||
Interface ratio | 1.26 | 0.12 | 3.47 | 0.38 | <0.0001 | |
Endometrial thickness (mm) | 1.26 | 0.06 | 0.68 | 0.04 | <0.0001 | |
Endometrial vessel area/total endometrial tissue area (%) | 3.92 | 0.51 | 11.44 | 1.31 | 0.0001 | |
Subepithelial capillaries/endometrial vessel area (%) | 1.13 | 0.29 | 24.52 | 5.31 | <0.0001 | |
Gland sections per 0.5 mm2 endometrium | 39 | 1.64 | 25 | 2.44 | <0.0001 | |
Glandular area/total endometrial tissue area (%) | 27.26 | 2.13 | 17.84 | 1.94 | 0.004 | |
Myometrial thickness (mm) | 0.99 | 0.05 | 0.96 | 0.03 | 0.37 |
Within the loosely packed middle part of the endometrium, tubular uterine glands exhibited narrow lumina with some cystically enlarged exceptions. Secretions were not present in any of the lumina. The uterine glandular epithelium consisted of columnar cells with a foamy cytoplasm and round to ovoid shaped nuclei located at the base of the cell. Uterine glandular epithelial cells showed protrusion reaching into the glandular lumen. Glands with little to no ciliated epithelial cells could be observed (Fig. 3a) as well as glands with abundant ciliated cells (Fig. 3b). The average number of uterine gland sections was 39 per 0.5 mm2 (s.e.m. = 1.64) which corresponded to 27.26% (s.e.m. = 2.13) of the total ROI endometrial area (Table 2).
Throughout the endometrium, intra- and extravascular immune cells were observed (not shown). Within the vessels, they often seemed to be attached to the endothelium. For further identification the implementation of specific immunohistological markers is planned in a subsequent study. Macrophage-like cells with foamy brown cytoplasm were often located in the vicinity of glandular ducts.
The smooth muscle cells of the myometrium formed an outer longitudinal and inner circular layer with a total height of 0.99 mm (s.e.m. = 0.05) (Table 2). Since the muscle fibers run from the outer to the inner region of the uterus in a tube-like structure and in a circular direction, the two layers could be hit several times in one cut. A blood vessel layer also referred to as Stratum vasculosum consisting of arteries and veins was located between the longitudinal and circular layer (Fig. 2a).
The perimetrium forming the outermost layer of the uterus was composed of an outer Tunica serosa comprised of a delicate line of squamous mesothelial cells with an underlying Tela subserosa, a thin layer of connective tissue including singular muscle fibers and a few capillaries (Fig. 2a).
Morphology of the gravid uterus
In the gravid uterus, the total height of the endometrium was 0.63 mm (s.e.m. = 0.04) which was significantly lower than in the non-gravid uterus (P < 0.0001) (Table 2).
The endometrium was folded and interdigitated with the folds formed by the embryonic BOM and TOM. Based on our calculations of the interface ratio between the luminal surface and the endometrial-myometrial interface, the mucosal folds caused a 2.75-fold increase (P < 0.0001) of the endometrial luminal epithelial surface in comparison to the non-gravid uterus. In most cases the folds were thin, highly vascularized extensions of stroma covered with epithelium. Only seldom they included glands. In most locations, the endometrial surface epithelium was separated from the embryonic yolk sac membranes (Fig. 4a).
Light micrographs of the gravid uterus at the time of birth stained with hematoxylin and eosin. Overview (a) and the embryo-maternal interface with the bilaminar omphalopleure in detail (b, c). c, subepithelial capillary; end, endoderm; em, endometrium; gl, uterine gland; le, luminal epithelium; mm, myometrium; smc, Stratum musculare circulare; sml, Stratum musculare longitudinale; pm, perimetrium; troph, trophoblast; v, blood vessel; green star, dense cell layer; yellow star, secretions.
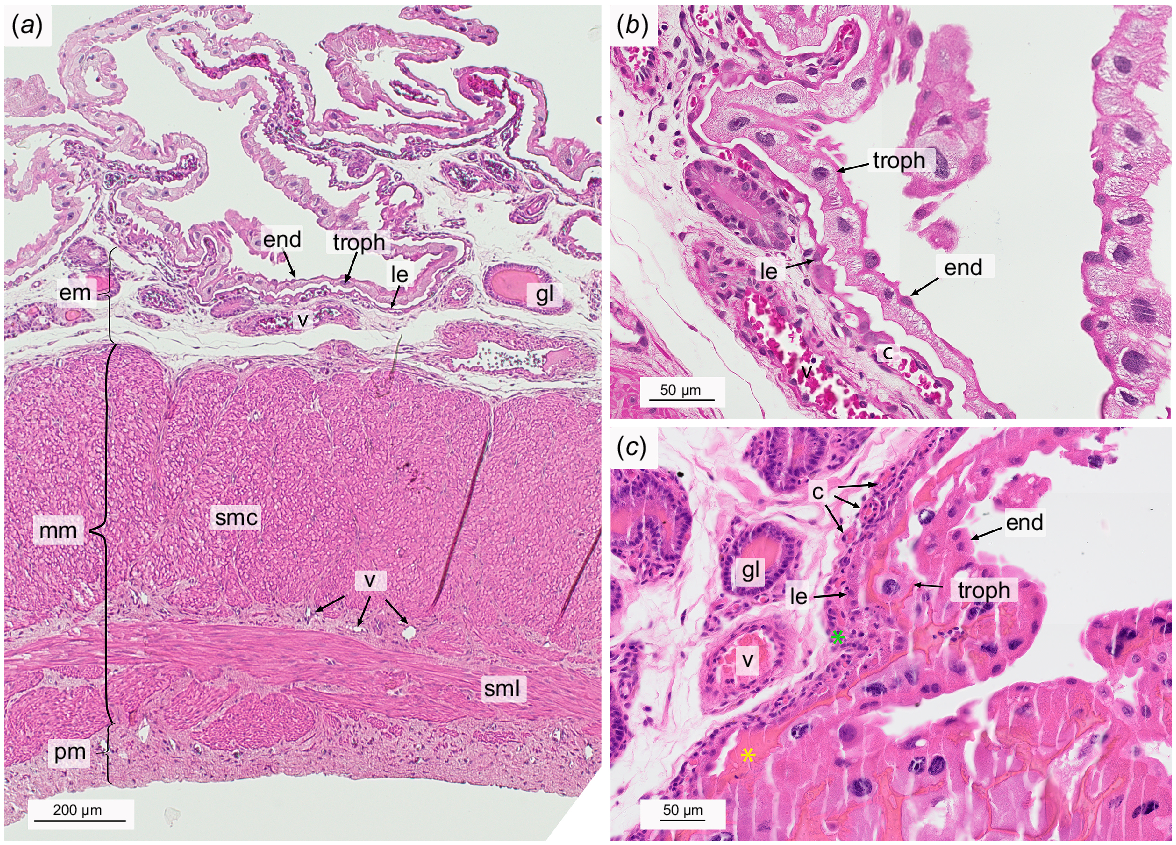
The cells of the Lamina epithelialis mucosae were dome-shaped or squamous and occasionally showed protrusions but it was not possible to distinguish between microvilli or kinocilia. No lymphocytes were visible within the luminal epithelium.
As in the non-gravid endometrium, the underlying Lamina propria mucosae could be separated into three layers: a less prominent dense subepithelial layer, a layer of loose connective tissue, and a layer containing large blood vessels towards the myometrium (Fig. 4a). The subepithelial capillaries had become much more pronounced compared to the non-gravid uterus (P < 0.0001), forming a dense network located right underneath the luminal epithelium (Fig. 4b). The cell body of the epithelial cells was stretched longitudinally and aligned parallel to the subepithelial capillaries lying closely below. Differentiation between the epithelial cells and the endothelial cells was almost impossible under the light microscope (Fig. 4b). 24.52% (s.e.m. = 5.31) of the endometrial blood vessel area was comprised by these subepithelial capillaries. In total the blood vessels made up 11.44% (s.e.m. = 1.31) of the endometrial area. This was significantly more than in the non-gravid endometrium (P = 0.0001) (Table 2).
The uterine glands generally had wider lumina than in the non-gravid endometrium, often filled with secretions (Fig. 4b, c). No cystically enlarged glands could be seen. The glandular epithelial cells were of columnar shape containing foamy cytoplasm and round to oval nuclei at the basal pole. Where the glandular lumen was not filled with secretions, cellular protrusions could be seen. 25 gland sections were counted per mm2 (s.e.m. = 2.44, P < 0.0001), which accounted for 17.84% (s.e.m. = 1.94) of the endometrial tissue (Figs 2, 4; Table 2). This was significantly less than in the non-gravid endometrium (P = 0.004) (Table 2). There was a moderate negative correlation (Schober et al. 2018) between the total vessel and the total gland area (r = −0.52).
As in the non-pregnant uterus, brownish macrophage-like cells were found close to the glands and associated capillaries.
Myometrial thickness was 0.96 mm (s.e.m. = 0.03) which was not significantly different to the non-gravid uterus (P = 0.37) (Table 2).
Morphology of the yolk sac placenta
The epitheliochorial yolk sac placenta was formed by the maternal luminal epithelium with its underlying subepithelial capillary network and the embryonic BOM and TOM. While BOM and TOM were closely apposed to the luminal epithelium in some parts, they were detached in others, but always following the winding folds of the maternal epithelium.
The bilaminar yolk sac consisted of two layers, namely the outer embryonic trophoblast and the endoderm lining the yolk sac cavity. The giant BOM trophoblast cells generally had large and frayed nuclei. Occasionally, only fragments of nuclei were present indicating cell decay. Other signs of degeneration of the BOM trophoblast giant cells included an irregular shape and vacuolized cytoplasm (Fig. 4b, c). The cell margins between the trophoblast cells appeared fringed where visible. The smaller cells of the endoderm exhibited a squamous shape with uneven lateral cell margins and their nuclei were small and round. Numerous binuclear endoderm cells could be detected (Fig. 4c). By means of Feulgen staining, we calculated an estimated average DNA content of 25 pg per trophoblast giant cell nuclei. However, the values were widely spread around the mean with a coefficient of variation (COV) of 44%. In comparison, the nuclei of the uterine epithelial cells had a haploid DNA content of 1C = 3.43 pg (COV = 15%).
The trilaminar portion of the yolk sac consisted of three parts, the trophectoderm, the endoderm and the vascularized mesenchyme spread out in between. The TOM trophoblast cells showed a squamous shape with an inhomogenous, strong eosinophilic cytoplasm and no visible cell margins. The nuclei were small, condensed and irregularly shaped. Embryonic capillaries contained nucleated embryonic erythrocytes. Occasionally, a few immune cells were encountered within the mesenchyme. The endoderm cells of the TOM were squamous with no visible lateral borders, confining the mesoderm towards the embryo. The cytoplasm expressed a fainter, inhomogeneous eosinophilic stain than in the BOM. The nuclei were round, small and sharply defined. (Fig. 5)
Discussion
With this study we provide the first quantification of endometrial blood vessels in general and subepithelial capillaries in particular in the gravid and non-gravid uterus of the tammar wallaby at the time of birth. Thereby, we contribute to a fundamental understanding of their functional differences. Likewise for the first time, we show the polyploidy of the giant BOM trophoblast cells.
We highlight the endometrial response to the embryo and suggest how the placental formation is highly efficient but likely self-limiting. This builds an important foundation for further studies regarding the embryo-maternal interactions eventually leading to parturition.
Comparison of endometrial thickness in gravid vs non-gravid uterus
The non-gravid and the gravid uterus differed in many aspects of their histomorphology. Our measurements showed that the endometrium of the gravid uterus was significantly thinner than the non-gravid endometrium. While a significantly thinner endometrium in the gravid compared to the non-gravid uterus was also observed in the distantly related virginia opossum, Didelphis virginiana, with a likewise non-invasive epitheliochorial yolk sac placenta (Fleming and Harder 1981), our results are in contrast to the study of Laird et al. (2016), where the thickness of the gravid and non-gravid endometrium (1.5 mm) in the tammar wallaby did not differ. The contradictory results might be explained by the extensive folding of the gravid endometrium. When measuring the endometrial thickness, we tried to measure up to the tip of the folds in a perpendicular line to the endometrial-myometrial interface. However, due to the winding of the endometrial folds, this was not always possible. Laird et al. (2016) do not provide any information on how they dealt with the endometrial folds regarding the measurements. As the extensive folding makes it difficult to get comparable results, endometrial thickness as such might not be a good parameter for histomorphologic comparison of the gravid and non-gravid uterus.
Increase in endometrial surface area
In fact, endometrial surface area might be a better parameter. We could show a significant 2.75-fold increase of the folded gravid endometrial surface compared to the non-gravid uterus. The folding extends the contact area between the embryonic trophoblast and the maternal epithelium ensuring efficient maternal-embryonic exchange. Increase of contact area is a common feature in placentation. Thereby, species with less invasive placenta types, such as ruminants or horses, usually show greater areas of interdigitation between embryonic and maternal tissue, whereas species with more invasive placenta types, such as primates or rodents, have more concentrated areas of direct contact (Amoroso 1952). In the pig’s epitheliochorial placenta an increase in endometrial folding throughout gestation has been positively correlated to an expansion of subepithelial capillaries (Seo et al. 2020) corresponding to what we could see in our study. As the tammar wallaby trophoblast does not invade the maternal tissue (Freyer et al. 2002), a strong increase in exchange area is necessary.
Cell rich subepithelial layer in the Lamina propria mucosae
In the non-gravid uterus, we observed a densely packed layer comprising of round shaped cells in the Lamina propria mucosae directly underlying the luminal epithelium. A similar layer was also present in the gravid endometrium, although less prominent.
In the opossum, these cells were identified as fibroblasts based on their cytoskeletal proteins (Kin et al. 2014). Proliferation of fibroblasts is enhanced by estrogens (Gerstenberg et al. 1999) and their key functions include extracellular matrix remodeling and tissue synthesis (Plikus et al. 2021). In species showing decidualization such as canines (Graubner et al. 2017) and humans (Liebig and Stegner 1977), these fibroblastic stromal cells differentiate first into predecidual and eventually into decidual stromal cells. Decidual cells are thought to provide immunological protection against embryonic semi-allograft and to regulate trophoblast invasion (Chavan et al. 2016).
In the tammar wallaby, no decidualization is observed (Wagner et al. 2014). Therefore, in the subepithelial layer of the gravid uterus fibroblasts and infiltrated immune cells might form a barrier to prevent the invasion of the trophectoderm. In the non-gravid uterus, the fibroblasts participate in the remodeling of the endometrium in preparation for pregnancy.
Vascularization and subepithelial capillaries
Although it has been demonstrated by histology (Freyer et al. 2002) and ultrasonography (Drews et al. 2013) that the blood flow increases in the gravid uterus, the vascularization has not yet been explicitly evaluated and quantified. We could show that three times more endometrial area of the gravid uterus was covered by blood vessels compared to the non-gravid uterus. This is very likely due to the presence of the embryo and the expression of vascular endothelial growth factor (VEGF) in its yolk sac placenta (Ager et al. 2008). VEGF has a strong angiogenic effect in the endometrial endothelial cells and promotes development of the subepithelial capillaries which push closely towards the embryo (Charnock-Jones et al. 2001; Freyer et al. 2002). We could show that these subepithelial capillaries accounted for almost 25% of the total blood vessel area of the gravid endometrium. In comparison, the proportion was only 1% for the non-gravid endometrium. This enormous increase in blood flow towards the endometrial surface underlines the importance of hemotrophic exchange in the late gestation of the tammar wallaby.
Comparison of endometrial gland profile in the gravid vs non-gravid uterus
The endometrium of the gravid uterus showed significantly fewer cross-sections of uterine glands per mm2 and a significantly smaller glandular area than the non-gravid uterus at this stage of gestation. A similar decrease in gland density has been shown in the distantly related Didelphidae gray short-tailed opossum, Monodelphis domestica, and virginia opossum, Didelphis virginiana. Both show a decrease in abundance of gland cross-sections in the second half of gestation (Fleming and Harder 1981). While in the gray short-tailed opossum this could be explained with the invasiveness of its placenta, the virginia opossum, like the tammar wallaby, has a non-invasive epitheliochorial yolk sac placenta (Freyer et al. 2003).
Contradictory to our quantification, Laird et al. (2016) counted almost twice as many cross-sections in the gravid (75/0.5 mm2) compared to the non-gravid endometrium (39/0.5 mm2). Unfortunately, Laird et al. (2016) only state the total number of animals sampled but not the number of samples per timepoint. To rule out the possibility that these contradictory results are due to individual differences, a re-evaluation with a larger sample size would be useful.
We showed that as the endometrial blood vessel area, especially the proportion of subepithelial capillaries, increased, the glandular area decreased. Consequently, the impact of histiotrophe seems to decline prior birth.
Embryo-maternal attachment and degeneration of the placenta preceding birth
Another focus of this study was set on the embryo-maternal interface. We could see an attachment between trophoblast and maternal epithelium only in a few places along the investigated embryo-maternal interface. In most BOM areas, the trophoblast cells were clearly separated from the maternal epithelium. In some areas, the space between the BOM trophoblast and the uterine luminal epithelium was filled with homogeneous secretion, most probably histiotrophe. Although it has been described that after implantation of the embryo on day 20 RPY, the microvilli of the trophoblast cells generally interdigitate with the maternal epithelial microvilli forming an intimate connection, a detachment prior to birth on day 26 RPY has likewise been observed by others (Denker and Tyndale-Biscoe 1986; Jones et al. 2014).
This detachment in the BOM area allows the trophoblast to immerse in the histotrophe accumulation between the maternal and embryonic interface enhancing nutrient transfer (Enders and Carter 2006). In return, the closer apposition in TOM, which contains the vascularized mesenchyme, results in a smaller distance to be bridged for hemoptrophic exchange.
Finally, the detachment might be a consequence of the observed decay in the BOM trophoblast cells indicating a degeneration of the placenta preceding birth.
Binuclear cells and regulation of placental growth
We observed binuclear endoderm cells at the time of birth in all of the BOM regions examined, but not in the TOM regions. Binuclear placental endoderm cells have been observed in the mouse (Ilgren 1980) and in the tammar wallaby between day 23 and 26 RPY (Jones et al. 2014).
In the liver, which is of endodermal origin, binuclearity is a common feature. There, the occurrence of binuclear hepatocytes is clearly associated with the end of the postnatal proliferative state eventually reducing DNA synthesis (Celton-Morizur and Desdouets 2010). In rats, the controlled failure of cytokinesis starts after weaning and is regulated by insulin (Tormos et al. 2015). In the tammar wallaby, insulin is strongly expressed in the yolk sac endoderm at days 23–24 RPY (Ager et al. 2007). Therefore, among other roles, the expression of insulin towards the end of gestation might lead to the suppression of cytokinesis in marsupial placental endoderm cells, leading to an increased binuclearity and thereby the regulation of placental growth.
Polyploidy of trophoblast giant cells
Trophoblast giant cells with enlarged nuclei have been described by Denker and Tyndale-Biscoe (1986) and Jones et al. (2014) throughout placentation. We demonstrate that these giant trophoblast cells are actually polyploid. Trophoblast giant cells have likewise been identified in the human (Zybina et al. 2002) many other species including the mink (Zybina et al. 1992), the mouse (Hunt and Avery 1971), and the alpaca (Klisch et al. 2006).
In those species polyploidy of these cells has been confirmed (Hunt and Avery 1971; Zybina et al. 1992; Klisch et al. 2006). By means of Feulgen staining we estimated a mean DNA content of 25 pg DNA per cell for the giant BOM trophoblast cells and a DNA content of 2C = 6.68 pg/cell for the uterine glandular epithelial cells. On average, this corresponds to a 7.6-fold DNA content in the BOM trophoblast cells. The result for the uterine glandular epithelial cell nuclei is in line with the results of the genome sequencing performed by the Vertebrates Genome Project in 2023 (1C = 3.4 Gb, National Center for Biotechnology Information 2023) and the Tammar Wallaby Genome Sequencing Consortium in 2009 (1C = 3.1 Gb, National Center for Biotechnology Information 2009).
We measured DNA contents of the trophoblast giant cell nuclei ranging from 12.37 pg/cell to 63.00 pg/cell. However, the nuclei presented different stages of polyploidy and, as mentioned earlier, we only evaluated sections of the nuclei. Therefore, we can state that the DNA content was higher than in the uterine glandular epithelial cells, indicating polyploidy, but only estimate to what degree of polyploidy this corresponds. This also accounts for the relatively high coefficient of variation (epithelial cell nuclei 11.81%, trophoblast cell nuclei 46.85%).
Polyploidy in trophoblast cells results from two mechanisms: endoreplication or endomitosis (Sarto et al. 1982; Zybina and Zybina 2005) which often occur in combination (Zybina and Zybina 2005). Endomitosis describes a disruption of the cell cycle in anaphase. As a result, the nucleus does not divide in telophase and cytokinesis does not occur. Thereafter, the cell contains a single giant, tetraploid nucleus (Zielke et al. 2013). A cell undergoing endoreplication circumvents mitosis and the following cytokinesis and instead undergoes several rounds of DNA-replication in S-phase, creating a single, enlarged, polyploid nucleus (Zielke et al. 2013).
As far as we can say from evaluating sections of the nuclei, the tammar wallaby trophoblast giant cells showed rather a low degree of polyploidy. Zybina and Zybina (2014) have related the degree of polyploidy to the degree of invasiveness. Species with highly invasive trophoblast cells show high rates of endoreplications whereas species with less invasive placenta types show low endomitosis rates (Klisch et al. 1999; Zybina et al. 2001; Zybina and Zybina 2005). Bearing in mind that the tammar wallaby has a non-invasive placenta (Freyer et al. 2002), this matches our findings.
The biological significance of polyploidy in extraembryonic tissues is still under debate (Hu and Cross 2010; Darmasaputra et al. 2024). One advantage of polyploidy is that it allows the placenta to grow fast and with little energy expenditure (Zybina and Zybina 2005; Hu and Cross 2010). We propose that in the tammar wallaby, the polyploidy of the giant trophoblast cells allows fast growth of the trophoblast at the beginning of the placentation period by omitting cell division. In this way, the trophoblast surface can keep up with the fast increase in endometrial surface area during the development of the yolk sac placenta. The increased surface area of polyploid trophoblast cells may enhance their ability to absorb as well as to phagocytose nutrients from the secretions they are surrounded with, which can then be transferred to the developing embryo. Another advantage of omitting the cell division is that the barrier towards the maternal tissue is never disrupted, maintaining the embryonic micro-environment (Darmasaputra et al. 2024). However, there is a strong correlation between polyploidy and loss of replicative potential (Darmasaputra et al. 2024). This suggests that similar to the binuclearity in the endoderm cells, the polyploidy of the giant BOM trophoblast cells eventually becomes a limiting factor for placental growth. Furthermore, it has been shown that the trophoblast giant cells in mice produce several angiogenic and vasodilatory factors such as VEGF and nitric oxide. This is essential for the modification of the endometrial vasculature to ensure sufficient blood flow towards the embryo (Hemberger 2008) and in the tammar wallaby could also lead to the observed increase in vascularization.
Degeneration of BOM trophoblast cells
The signs of decay in the BOM giant cells we observed at the time of birth (day 26 RPY) have likewise been seen by Jones et al. (2014) on day 23 RPY via electron microscopy and by Denker and Tyndale-Biscoe (1986) on day 26 RPY but not yet on day 22 RPY.
While mammals with a considerably longer pregnancy such as the human (Zybina et al. 2002), the silver fox (Zybina et al. 2001) or the cow (Klisch et al. 1999) retain a portion of diploid trophoblast cells for proliferation of the placenta, we found polyploid and degenerating trophoblast cells throughout the whole bilaminar area of the placenta of the tammar wallaby. The combination of degenerating trophoblast cells and the lack of diploid cells for continuous proliferation supports the hypothesis that the placenta reaches its limits and becomes unable to fulfill the embryo’s needs making birth inevitable for the survival of the young.
In a recent study, Dudley et al. (2024) showed that while the tammar wallaby reacts only with a moderate inflammatory response to the attachment of the embryo, there is an overrepresentation of genes prior to birth associated with inflammation, such as interleukins and their receptors, genes associated with prostaglandin synthesis and signaling, and genes involved in neutrophile activation. We therefore suggest that, while the trophoblast giant cells might help to modify the maternal inflammation response in late gestation, the final degeneration of those cells at the time of birth could contribute to a maternal inflammatory reaction (Hansen et al. 2017; Stadtmauer and Wagner 2020) supporting the induction of birth.
Limitations and scope
It was striking to see the drastic increase in subepithelial capillary area and how the glandular area negatively correlates with the endometrial blood vessel area. To test our hypothesis that hemotrophe and vascular exchanges become more important in late gestation than nutrient exchange via histiotrophe, it would be interesting to quantify the endometrial blood vessels and the subepithelial capillaries as well as the glandular area at several time points after implantation up until birth.
An investigation of the abundance of polyploid trophoblast giant cells and binuclear endoderm cells not only at parturition but also at different timepoints could support the self-limiting nature of placental growth. Furthermore, an immunohistochemical evaluation and quantification of apoptosis in the BOM trophoblast giant cells would give further insight into the degenerative processes in the yolk sac.
In the stroma of the non-gravid endometrium as well as of the gravid endometrium we found a variance of immune cells. These are presumably involved in the maternal allo-recognition of the embryo and need to be further analyzed.
Lastly, it should not remain unmentioned that the use of indomethacin to prevent birth could impact some of the results. There is not much information on how the COX-inhibitor affects the wallaby uterus other than that it cuts the release of prostaglandins preventing birth as well as a decline of progesterone before parturition (Renfree et al. 1994). While interpreting the vascularization in our samples, one should keep in mind that the inhibition of prostaglandins has a restraining effect on VEGFR2 and hence on the development of the uterine vessels (Tamura et al. 2006). Nevertheless, it underlines the striking increase in blood vessel area in the gravid uterus even without the stimulating impact of VEGFR2 and prostaglandin E2.
Although it is known that prostaglandins have a positive impact on the adhesion of porcine trophoblast cells to the maternal epithelial cells (Waclawik et al. 2013), a separation between endometrium and trophoblast like we observed in our samples was similarly described in other studies on wallabies that have not been treated with indomethacin (Denker and Tyndale-Biscoe 1986; Jones et al. 2014). These other studies likewise found a degeneration of the BOM trophoblast giant cells. Thus, we argue that both events are very likely to occur independently from the treatment and are therefore valid observations.
Conclusion
In conclusion, our study points out significant differences in the endometrium of the non-gravid and gravid uterus at the time of birth of the tammar wallaby. We highlight how the embryo-maternal interface has adapted to ensure the rapid growth of the embryo. The confirmed polyploidy of the BOM trophoblast giant cells indicate their importance in the rapid growth of the placenta and in providing sufficient nutrient exchange. The binuclearity of the endoderm cells towards the end of gestation in combination with the polyploidy of the BOM trophoblast giant cells seem to be the limiting factors when it comes to prolonging intrauterine life. With the degeneration of the polyploid trophoblast cells, the dying cells might trigger a sterile inflammatory immune response leading to birth. The highlighted adaptations of the endometrium and the embryonic part suggest that this placental strategy is not only optimized for brief intra-uterine development but actually prevents prolonged gestation. This ensures the female is ready to seize the ovulation of the contralateral ovary to ensure that a new pregnancy is already in progress when the pouch becomes available again. This maximizes reproductive success in unpredictable environments during the short reproductive season.
Data availability
The data that supports this study will be shared upon reasonable request to the corresponding author.
Acknowledgements
The authors would like to thank Prof. Marilyn Renfree for generously providing samples, Gabriela Vorburger and Veronique Gaschen for their excellent support in the laboratory, Alexander Ernst from Nikon Europe for his advice on the work with the artificial intelligence, Dr. Laura Cunha Silva and Dr. Beatriz Vidondo for statistical consulting and Michelle Gygax for sharing her expertise in the use of RStudio and Photoshop.
References
Ager E, Suzuki S, Pask A, Shaw G, Ishino F, Renfree MB (2007) Insulin is imprinted in the placenta of the marsupial, Macropus eugenii. Developmental Biology 309(2), 317-328.
| Crossref | Google Scholar | PubMed |
Ager EI, Pask AJ, Shaw G, Renfree MB (2008) Expression and protein localisation of IGF2 in the marsupial placenta. BMC Developmental Biology 8(1), 17.
| Crossref | Google Scholar |
Buentjen I, Drews B, Frankenberg SR, Hildebrandt TB, Renfree MB, Menzies BR (2015) Characterisation of major histocompatibility complex class I genes at the fetal-maternal interface of marsupials. Immunogenetics 67(7), 385-393.
| Crossref | Google Scholar | PubMed |
Celton-Morizur S, Desdouets C (2010) Polyploidization of liver cells. In ‘Polyploidization and Cancer’. (Ed. RYC Poon) pp. 123–135. (Springer New York: New York, NY, USA) 10.1007/978-1-4419-6199-0_8
Charnock-Jones DS, Clark DE, Licence D, Day K, Wooding FB, Smith SK (2001) Distribution of vascular endothelial growth factor (VEGF) and its binding sites at the maternal-fetal interface during gestation in pigs. Reproduction 122(5), 753-760.
| Crossref | Google Scholar | PubMed |
Chavan AR, Bhullar B-AS, Wagner GP (2016) What was the ancestral function of decidual stromal cells? A model for the evolution of eutherian pregnancy. Placenta 40, 40-51.
| Crossref | Google Scholar | PubMed |
Cruz YP, Selwood L (1997) Histological differences between gravid and non-gravid uteri in the dasyurid marsupial, Sminthopsis macroura (Spencer). Reproduction 111(2), 319-325.
| Crossref | Google Scholar |
Darmasaputra GS, van Rijnberk LM, Galli M (2024) Functional consequences of somatic polyploidy in development. Development 151, dev202392.
| Crossref | Google Scholar |
Denker HW, Tyndale-Biscoe CH (1986) Embryo implantation and proteinase activities in a marsupial (Macropus eugenii). Cell and Tissue Research 246, 279-291.
| Crossref | Google Scholar |
Drews B, Roellig K, Menzies BR, Shaw G, Buentjen I, Herbert CA, Hildebrandt TB, Renfree MB (2013) Ultrasonography of wallaby prenatal development shows that the climb to the pouch begins in utero. Scientific Reports 3, 1458.
| Crossref | Google Scholar |
Dudley JS, Renfree MB, Wagner GP, Griffith OW (2024) The extension of mammalian pregnancy required taming inflammation: independent evolution of extended placentation in the tammar wallaby. Proceedings of the National Academy of Sciences of the United States of America 121(42), e2310047121.
| Crossref | Google Scholar |
Enders AC, Carter AM (2006) Comparative placentation: some interesting modifications for histotrophic nutrition – a review. Placenta 27, 11-16.
| Crossref | Google Scholar |
Fleming MW, Harder JD (1981) Uterine histology and reproductive cycles in pregnant and non-pregnant opossums, Didelphis virginiana. Journal of Reproduction and Fertility 63(1), 21-24.
| Crossref | Google Scholar | PubMed |
Freyer C, Zeller U, Renfree MB (2002) Ultrastructure of the placenta of the tammar wallaby, Macropus eugenii: comparison with the grey short-tailed opossum, Monodelphis domestica. Journal of Anatomy 201(2), 101-119.
| Crossref | Google Scholar |
Freyer C, Zeller U, Renfree MB (2003) The marsupial placenta: a phylogenetic analysis. Journal of Experimental Zoology Part A: Comparative Experimental Biology 299(1), 59-77.
| Crossref | Google Scholar | PubMed |
Gerstenberg C, Allen WR, Stewart F (1999) Cell proliferation patterns in the equine endometrium throughout the non-pregnant reproductive cycle. Reproduction 116(1), 167-175.
| Crossref | Google Scholar |
Gordon K, Fletcher TP, Renfree MB (1988) Reactivation of the quiescent corpus luteum and diapausing embryo after temporary removal of the sucking stimulus in the tammar wallaby (Macropus eugenii). Journal of Reproduction and Fertility 83(1), 401-406.
| Crossref | Google Scholar | PubMed |
Graubner FR, Reichler IM, Rahman NA, Payan-Carreira R, Boos A, Kowalewski M (2017) Decidualization of the canine uterus: from early until late gestational in vivo morphological observations, and functional characterization of immortalized canine uterine stromal cell lines. Reproduction in Domestic Animals 52(S2), 137-147.
| Crossref | Google Scholar |
Gregory TR (2024) Animal genome size database. Available at http://www.genomesize.com
Hansen VL, Faber LS, Salehpoor AA, Miller RD (2017) A pronounced uterine pro-inflammatory response at parturition is an ancient feature in mammals. Proceedings of the Royal Society B: Biological Sciences 284(1865), 20171694.
| Crossref | Google Scholar |
Hardie DC, Gregory TR, Hebert PDN (2002) From pixels to picograms. Journal of Histochemistry & Cytochemistry 50(6), 735-749.
| Crossref | Google Scholar | PubMed |
Hemberger M (2008) IFPA Award in placentology lecture – characteristics and significance of trophoblast giant cells. Placenta 29, 4-9.
| Crossref | Google Scholar |
Hickford D, Shaw G, Renfree MB (2008) In vitro culture of peri-gastrulation embryos of a macropodid marsupial. Journal of Anatomy 212(2), 180-191.
| Crossref | Google Scholar | PubMed |
Hinds LA, Tyndale-Biscoe CH (1982) Plasma progesterone levels in the pregnant and non-pregnant tammar, Macropus eugenii. Journal of Endocrinology 93(1), 99-107.
| Crossref | Google Scholar | PubMed |
Hu D, Cross JC (2010) Development and function of trophoblast giant cells in the rodent placenta. International Journal of Developmental Biology 54(2–3), 341-354.
| Crossref | Google Scholar | PubMed |
Hunt CV, Avery GB (1971) Increased levels of deoxyribonucleic acid during trophoblast giant-cell formation in mice. Reproduction 25(1), 85-91.
| Crossref | Google Scholar |
Ilgren EB (1980) Polyploidization of extraembryonic tissues during mouse embryogenesis. Development 59(1), 103-111.
| Crossref | Google Scholar |
Jones CJP, Skepper JN, Renfree MB, Aplin JD (2014) Trophoblast specialisations during pregnancy in the tammar wallaby, Macropus eugenii: a morphological and lectin histochemical study. Placenta 35(7), 467-475.
| Crossref | Google Scholar | PubMed |
Kin K, Maziarz J, Wagner GP (2014) Immunohistological study of the endometrial stromal fibroblasts in the opossum, Monodelphis domestica: evidence for homology with eutherian stromal fibroblasts. Biology of Reproduction 90(5), 111 1-12.
| Crossref | Google Scholar |
Klisch K, Hecht W, Pfarrer C, Schuler G, Hoffmann B, Leiser R (1999) DNA content and ploidy level of bovine placentomal trophoblast giant cells. Placenta 20(5–6), 451-458.
| Crossref | Google Scholar | PubMed |
Klisch K, Bevilacqua E, Olivera LVM (2006) Mitotic polyploidization in trophoblast giant cells of the alpaca. Cells Tissues Organs 181(2), 103-108.
| Crossref | Google Scholar | PubMed |
Laird MK, Hearn CM, Shaw G, Renfree MB (2016) Uterine morphology during diapause and early pregnancy in the tammar wallaby (Macropus eugenii). Journal of Anatomy 229(3), 459-472.
| Crossref | Google Scholar | PubMed |
Laird MK, Dargan JR, Paterson L, Murphy CR, McAllan BM, Shaw G, Renfree MB, Thompson MB (2017) Uterine molecular changes for non-invasive embryonic attachment in the marsupials Macropus eugenii (Macropodidae) and Trichosurus vulpecula (Phalangeridae). Molecular Reproduction and Development 84(10), 1076-1085.
| Crossref | Google Scholar | PubMed |
Liebig W, Stegner H-E (1977) Die Dezidualisation der endometrialen Stromazelle. Archiv Für Gynäkologie 223(1), 19-31.
| Crossref | Google Scholar |
Merchant JC (1979) The effect of pregnancy on the interval between one oestrus and the next in the tammar wallaby, Macropus eugenii. Journal of Reproduction and Fertility 56(2), 459-463.
| Crossref | Google Scholar | PubMed |
National Center for Biotechnology Information (2009) Genome - GCA_000004035.1. National Institutes of Health. Available at https://www.ncbi.nlm.nih.gov/datasets/genome/GCA_000004035.1/ [accessed 23 April 2024]
National Center for Biotechnology Information (2023) Genome - GCA_028372415.1. National Institutes of Health. Available at https://www.ncbi.nlm.nih.gov/datasets/genome/GCA_028372415.1/ [accessed 23 April 2024]
NCSS 2024 Statistical Software (2024) NCSS, LLC, Kaysville, Utah, USA. Available at https://www.ncss.com/software/ncss/
Plikus MV, Wang X, Sinha S, Forte E, Thompson SM, Herzog EL, Driskell RR, Rosenthal N, Biernaskie J, Horsley V (2021) Fibroblasts: origins, definitions, and functions in health and disease. Cell 184(15), 3852-3872.
| Crossref | Google Scholar | PubMed |
R Core Team (2022) ‘R: A language and environment for statistical computing.’ (R Foundation for Statistical Computing: Vienna, Austria) Available at https://www.R-project.org/
Renfree MB (1973) The composition of fetal fluids of the marsupial Macropus eugenii. Developmental Biology 33(1), 62-79.
| Crossref | Google Scholar | PubMed |
Renfree MB, Lewis AM (1996) Cleavage in vivo and in vitro in the marsupial Macropus eugenii. Reproduction, Fertility and Development 8(4), 725-742.
| Crossref | Google Scholar | PubMed |
Renfree MB, Shaw G, Fletcher TP (1994) Evidence for the essential role of prostaglandins for parturition in a marsupial, Macropus eugenii. Reproduction 102(2), 433-446.
| Crossref | Google Scholar |
Renfree MB, Papenfuss AT, Deakin JE, Lindsay J, Heider T, Belov K, Rens W, Waters PD, Pharo EA, Shaw G, Wong ESW, Lefèvre CM, Nicholas KR, Kuroki Y, Wakefield MJ, Zenger KR, Wang C, Ferguson-Smith M, Nicholas FW, Hickford D, Yu H, Short KR, Siddle HV, Frankenberg SR, Chew KY, Menzies BR, Stringer JM, Suzuki S, Hore TA, Delbridge ML, Mohammadi A, Schneider NY, Hu Y, O’Hara W, Al Nadaf S, Wu C, Feng ZP, Cocks BG, Wang J, Flicek P, Searle SMJ, Fairley S, Beal K, Herrero J, Carone DM, Suzuki Y, Sugano S, Toyoda A, Sakaki Y, Kondo S, Nishida Y, Tatsumoto S, Mandiou I, Hsu A, McColl KA, Lansdell B, Weinstock G, Kuczek E, McGrath A, Wilson P, Men A, Hazar-Rethinam M, Hall A, Davis J, Wood D, Williams S, Sundaravadanam Y, Muzny DM, Jhangiani SN, Lewis LR, Morgan MB, Okwuonu GO, Ruiz SJ, Santibanez J, Nazareth L, Cree A, Fowler G, Kovar CL, Dinh HH, Joshi V, Jing C, Lara F, Thornton R, Chen L, Deng J, Liu Y, Shen JY, Song XZ, Edson J, Troon C, Thomas D, Stephens A, Yapa L, Levchenko T, Gibbs RA, Cooper DW, Speed TP, Fujiyama A, Graves JAM, O’Neill RJ, Pask AJ, Forrest SM, Worley KC (2011) Genome sequence of an Australian kangaroo, Macropus eugenii, provides insight into the evolution of mammalian reproduction and development. Genome Biology 12(8), R81.
| Crossref | Google Scholar |
Sarto F, Cominato I, Bianchi V, Levis AG (1982) Increased incidence of chromosomal aberrations and sister chromatid exchanges in workers exposed to chromic acid (CrO3) in electroplating factories. Carcinogenesis 3(9), 1011-1016.
| Crossref | Google Scholar | PubMed |
Schober P, Boer C, Schwarte L (2018) Correlation coefficients: appropriate use and interpretation. Anesthesia & Analgesia 126(5), 1763-1768.
| Crossref | Google Scholar | PubMed |
Seo H, Li X, Wu G, Bazer FW, Burghardt RC, Bayless KJ, Johnson GA (2020) Mechanotransduction drives morphogenesis to develop folding during placental development in pigs. Placenta 90, 62-70.
| Crossref | Google Scholar | PubMed |
Stadtmauer DJ, Wagner GP (2020) Cooperative inflammation: The recruitment of inflammatory signaling in marsupial and eutherian pregnancy. Journal of Reproductive Immunology 137, 102626.
| Crossref | Google Scholar | PubMed |
Tamura K, Sakurai T, Kogo H (2006) Relationship between prostaglandin E2 and vascular endothelial growth factor (VEGF) in angiogenesis in human vascular endothelial cells. Vascular Pharmacology 44(6), 411-416.
| Crossref | Google Scholar | PubMed |
Tormos AM, Taléns-Visconti R, Sastre J (2015) Regulation of cytokinesis and its clinical significance. Critical Reviews in Clinical Laboratory Sciences 52(4), 159-167.
| Crossref | Google Scholar | PubMed |
Tyndale-Biscoe H, Renfree M (1987) Pregnancy and parturition. In ‘Reproductive physiology of marsupials’. pp. 258–342. (Cambridge University Press) 10.1017/CBO9780511623493.008
Waclawik A, Kaczynski P, Jabbour HN (2013) Autocrine and paracrine mechanisms of prostaglandin E2 action on trophoblast/conceptus cells through the prostaglandin E2 receptor (PTGER2) during implantation. Endocrinology 154(10), 3864-3876.
| Crossref | Google Scholar | PubMed |
Wagner GP, Kin K, Muglia L, Pavličev M (2014) Evolution of mammalian pregnancy and the origin of the decidual stromal cell. International Journal of Developmental Biology 58, 117-126.
| Crossref | Google Scholar | PubMed |
Zielke N, Edgar BA, DePamphilis ML (2013) Endoreplication. Cold Spring Harbor Perspectives in Biology 5(1), a012948.
| Crossref | Google Scholar | PubMed |
Zybina TG, Zybina EV (2005) Cell reproduction and genome multiplication in the proliferative and invasive trophoblast cell populations of mammalian placenta. Cell Biology International 29(12), 1071-1083.
| Crossref | Google Scholar | PubMed |
Zybina TG, Zybina EV (2014) Genome variation in the trophoblast cell lifespan: diploidy, polyteny, depolytenization, genome segregation. World Journal of Medical Genetics 4(4), 77-93.
| Crossref | Google Scholar |
Zybina EV, Zybina TG, Isakova GK, Kiknadze II, Shteĭn GI (1992) [Polyploidy and polyteny in the trophoblast cells of the mink]. Tsitologiia 34(11–12), 55-59.
| Google Scholar | PubMed |
Zybina TG, Zybina EV, Kiknadze II, Zhelezova AI (2001) Polyploidization in the trophoblast and uterine glandular epithelium of the endotheliochorial placenta of silver fox (Vulpes fulvus Desm.), as revealed by the DNA content. Placenta 22(5), 490-498.
| Crossref | Google Scholar | PubMed |
Zybina TG, Kaufmann P, Frank HG, Freed J, Kadyrov M, Biesterfeld S (2002) Genome multiplication of extravillous trophoblast cells in human placenta in the course of differentiation and invasion into endometrium and myometrium. I. Dynamics of polyploidization. Tsitologiia 44(11), 1058-1067.
| Google Scholar | PubMed |
Zybina EV, Bogdanova MS, Stein GI, Vlasova TD, Zybina TG (2009) Endopolyploidization and the interstitial invasion of the supergiant trophoblast cells of the field vole Microtus rossiaemeridionalis. Tissue and Cell 41(5), 362-366.
| Crossref | Google Scholar | PubMed |