Constraining nitrogen sources to a seagrass-dominated coastal embayment by using an isotope mass balance approach
Douglas G. Russell A , Adam J. Kessler

A Water Studies Centre, School of Chemistry, Monash University, Clayton, Vic. 3800, Australia.
B School of Earth, Atmosphere and Environment, Monash University, Clayton, Vic. 3800, Australia.
C Department of Estuarine and Delta Systems, Royal Netherlands Institute for Sea Research (NIOZ-Yerseke), Yerseke, Netherlands.
Marine and Freshwater Research 73(5) 703-709 https://doi.org/10.1071/MF21320
Submitted: 9 November 2021 Accepted: 17 January 2022 Published: 25 February 2022
© 2022 The Author(s) (or their employer(s)). Published by CSIRO Publishing. This is an open access article distributed under the Creative Commons Attribution-NonCommercial-NoDerivatives 4.0 International License (CC BY-NC-ND)
Abstract
Nitrogen (N) is often the key nutrient limiting primary production in coastal waters. Quantifying sources and sinks of N is therefore critical to understanding the factors that underpin the productivity of coastal ecosystems. Constraining nitrogen inputs can be difficult for some terms such as N fixation and marine exchange as a consequence of uncertainties associated with scaling and stochasticity. To help overcome these issues, we undertook a N budget incorporating an isotope and mass balance to constrain N sources in a large oligotrophic coastal embayment (Western Port, Australia). The total N input to Western Port was calculated to be 1400 Mg N year−1, which is remarkably consistent with previous estimates of sedimentation rates within the system. Catchment inputs, N fixation, marine sources and atmospheric deposition comprised 44, 28, 28 and 13% of N inputs respectively. Retention of marine-derived N equated to ~3 and ~10% of total N and NOx flushed through the system from the marine end-member. The relatively high contribution of N fixation compared with previous studies was most likely to be due to the high proportion of nutrient-limited intertidal sediments where N is mediated by seagrasses and sediment cyanobacteria.
Keywords: 15N, denitrification, isotope, nitrogen, nitrogen budget, nitrogen fixation, seagrass, tidal flat.
Introduction
Nitrogen inputs to the coastal zone are one of the most pressing issues facing the biosphere (Steffen et al. 2015). Excessive nitrogen loads have led to algal blooms, hypoxia and seagrass loss in the coastal zone (Rabalais et al. 2002; Cook and Holland 2012; Cook et al. 2015). Anthropogenic sources of nitrogen enter via rivers, atmospheric deposition and sewage outfalls. In addition to anthropogenic sources, nitrogen can enter the coastal zone from offshore marine sources and nitrogen fixation. Nitrogen budgets are very commonly used to quantify the relative importance of these sources. For example, in Moreton Bay, Australia, it was found that nitrogen fixation was the dominant nitrogen input, being approximately double that of the catchment inputs (Eyre and Mc Kee 2002). By contrast, Chesapeake Bay nitrogen inputs are dominated by rivers, with nitrogen inputs by nitrogen fixation being considered negligible (Boynton et al. 1995), and in Golden and Tasman Bay in New Zealand, inputs were dominated by the marine source (Zeldis and Swaney 2018).
The reliability of nitrogen budgets will depend on how well constrained the input terms are. In reality, there will always be terms that are difficult to constrain depending on the geomorphology of the system and the availability of monitoring data. The accuracy of riverine inputs is dependent on the intensity of monitoring data during flow events (Harmel et al. 2009) and atmospheric deposition can have highly variable concentrations of nitrate depending on air source (Eriksson 1952). Marine exchange of nitrogen is particularly challenging to quantify, given the large, and hard to constrain, volumes of water exchanged within marine systems. As a consequence, it is generally assumed that marine exchange is the difference between inputs and internal sinks (Boynton et al. 1995; Eyre and Mc Kee 2002), which is highly uncertain. Where a salinity gradient exists, salt budgets can be used to infer inputs from terrestrial and marine end-members (Gordon et al. 1996); however, this gives only a measure of an instantaneous input, leading to a high degree of uncertainty in scaling this over time.
Internal nitrogen addition and removal processes, including denitrification and nitrogen fixation, further add to the complexity of nitrogen budgets. Measurements of these process rates suffer from two key issues, including the accuracy of the rate measurement itself and scaling. With regard to the first issue, enclosure of the system may change metabolic and transport processes that can potentially change rates through the disruption of faunal and plant integrity. Furthermore, methods to measure denitrification and nitrogen fixation have several assumptions and potential shortcomings associated with tracer additions. For denitrification measured using the isotope pairing technique, it is assumed that the 15NO3− added mixes homogenously into all denitrification zones, which might not always be the case in vegetated sediments (Risgaard-Petersen and Jensen 1997). In the case of nitrogen fixation, there are also assumptions about the conversion ratio of acetylene to ethylene when using the acetylene reduction assay, or the homogeneity of mixing of 15N2 into the zones of nitrogen fixation when using the 15N labelling approach (Capone and Kiene 1988; Karl et al. 2002)
With regard to the second issue, the extrapolation of nitrogen fixation and denitrification rates from discrete measurements to larger spatial scales, even in the case of local studies, can also account for the variability in the relative importance of these processes considered in previous studies. For instance, Mulholland (2007) found that a wide range of global rates of nitrogen fixation can be obtained, depending on whether the measurements are extrapolated from laboratory- or field-based experiments. Furthermore, the temporal and spatial variability of these processes means that repeated measurements over many different environments are required to get a truly representative estimation of these processes across time and space.
The use of stable isotopes offers a potential solution to the problems described above that use a mass balance only approach. The most easily constrained systems are those with limited nitrogen end-members such as the open ocean (Mahaffey et al. 2003). This approach has also been used in more complex coastal systems (Liu et al. 1996; Voss et al. 2005; Radtke et al. 2012; Korth et al. 2014; Woodland and Cook 2014); however, the focus has been on constraining nitrogen fixation in pelagic systems where blooms of cyanobacteria are known to occur. Currently, there have been no studies using isotope mass balance approaches to constrain nitrogen budgets in coastal embayments. These systems are more complex than open ocean systems that have been previously studied; however, if the isotope end-members are well constrained, then adding isotopes into the mass balance can help constrain the nitrogen budget. Riverine nitrogen isotope signatures are highly variable depending on the land-use types, with δ15N values increasing from ~4 to >20‰ as land-use intensity increases from forested to agricultural catchments (Wong et al. 2018). Atmospheric deposition is typically <0‰, although this can be highly variable (Proemse et al. 2013). The marine δ15N end-member typically ranges between 6 and 10‰ in the surface oceans globally (Rafter et al. 2019). Newly fixed nitrogen typically has a value of ~−2–0‰ (Owens 1988). The efficacy of adding δ15N into a nitrogen budget will therefore depend on the nature of a system. Systems likely to benefit most from the addition of δ15N onto the nitrogen budget are those where the putative dominant inputs have a distinct isotopic separation (for example, nitrogen fixation and rivers with a high δ15N or sewage). For example in the case of Moreton Bay (Eyre and Mc Kee 2002), δ15N would aid in validating the estimated high nitrogen fixation term relative to marine and catchment sources.
Here, we undertook a mass and stable isotope balance to create a nitrogen budget for a shallow embayment with a large marine exchange, Western Port, Australia. The aim was to better constrain marine nitrogen inputs to the system by using a novel isotope mass balance approach.
Materials and methods
Study site
The combined mass and isotope balance described in this study was undertaken across Western Port, a macrotidal shallow marine embayment of ~650 km2, 55 km south-east of Melbourne. The major tributaries flowing into the bay are Bass River, Bunyip River, Lang Lang River, Toomuc Creek and Watsons Creek, and there are two separate openings to the ocean located on either side of Phillip Island (Fig. 1, Table 1).
![]() |
![]() |
Atmospheric deposition
All budget mass and isotopic input terms are summarised in Table 2. Long-term rainfall data for the period 2006–2016 were obtained for Melbourne and were then extrapolated to the entire area of Western Port (https://www.melbournewater.com.au/water/rainfall-and-river-levels#/, accessed February 2017). On the basis of the previous work of Lansdown (2009) and Wong et al. (2014), it was assumed that the concentrations of NH4+ and NOx in the rainfall were 14 ± 1.4 and 14 ± 0.7 μmol L−1 respectively, resulting in an estimated total input of ~186 ± 20 tonnes (Mg) of nitrogen per year. Similarly, it was assumed that the end-members for the atmospheric deposition of NH4+ and NOx were 1.0 ± 2.0 and −1.4 ± 2.9‰ respectively, on the basis of the previous work of Lansdown (2009) and Wong et al. (2014).
Nitrogen fixation
The production of bioavailable nitrogen through nitrogen fixation was estimated to be 434 ± 40 Mg N year−1 on the basis of work by Russell et al. (2016). This value was obtained by extrapolating rates from vegetated and non-vegetated core incubations collected at Corinella, Coronet Bay and Rhyll (Fig. 1) throughout the austral winter–summer period (July 2014–March 2015). On the basis of the available literature, it was assumed that the isotopic end-member for nitrogen fixation was 0.0 ± 1.0‰ (Owens 1988).
Riverine inputs
The catchments and tributaries that surround Western Port also make a significant contribution of nitrogen input to the bay, with nitrogen entering in various forms, including total and dissolved nitrogen (in particular nitrate, NO3−). Nutrient concentrations for both total nitrogen (TN) and NO3− in addition to flow data for the major tributaries were available for the period 1990–2013 from the Department of Environment, Land, Water & Planning (http://data.water.vic.gov.au/monitoring.htm, accessed January 2017). Loading calculations were made using the GUMLEAF program (‘Generator Uncertainty Measures and Load Estimates using Alternative Formulae’, ver. 0.1 α, University of Melbourne, see https://environmetrics.net/resources/software/; Tan et al. 2005) in conjunction with the flow regime-stratified Kendall’s ratio estimator (Tan et al. 2005). Loads were calculated using daily flow data in combination with water-quality samples for each tributary collected, on average, in monthly intervals, and the average concentrations of NOx and TN were 6.48 and 7.9 mg L−1 respectively, and it was estimated that yearly input of total nitrogen was ~612 ± 30 Mg N year−1 (Russell et al. 2016).
The isotopic signatures (δ15N) of the catchment-derived NO3− flowing into the bay were taken from a previous study (Wong et al. 2018). During the present study, water samples were collected from the main tributaries flowing into Western Port between April 2014 and May 2015. These samples were collected from the most downstream freshwater section of each river or creek (Table 1), to ensure that all samples collected were terrestrially derived and not influenced by tidal exchange. Samples were filtered through 0.22-µm Sartorius Minisart syringe filters and frozen until analysis. The approach of McIlvin and Altabet (2005) was used to determine the isotopic signature of 15N–NO3−. Initially, cadmium was used to reduce NO3− to NO2−, afterwards sodium azide in an acetic acid buffer was used to further reduce the NO2− to N2O. The N2O produced was analysed on a Hydra 20-22 continuous-flow isotope ratio mass spectrometer (CF-IRMS; Sercon Ltd, UK) interfaced to a cryoprep system (Sercon Ltd, UK); the precision of the stable isotope analysis was ±0.3‰ (s.d.; n = 5) for NO3− concentration >5 µmol L−1.
![]() |
The isotopic signature of the total nitrogen load was estimated using average isotopic signature of particulate (SPN) and dissolved (NO3−) nitrogen weighted for their respective loads. Samples of SPN (n = 30; November 2015–October 2016) were collected under high and low flow conditions to ensure that the riverine isotopic signature estimated was representative of the annual input of riverine nitrogen into Western Port. Only concentrations and isotopes of NOx and particulate nitrogen were considered when calculating the total nitrogen loads. The fractions of NH4+ and dissolved organic nitrogen were ignored, on the basis that these fractions generally comprised <5 and <10% of total nitrogen respectively. Pre-ashed Whatmann 25 mm GF/F paper was used to determine the isotopic signature of the particulate nitrogen. These filters were then dried to a constant weight at 60°C for 48 h, before being analysed using an ANCA GSL2 elemental analyser interfaced to a Hydra 20–22 continuous-flow isotope ratio mass-spectrometer (Sercon Ltd, UK). Stable isotope data for nitrogen were reported in delta notation (Eqn 1) relative to the 15N:14N ratio of air, the precision of the stable isotope analysis was ±0.2‰ (s.d.; n = 5) and the precision of the elemental analysis was ± 0.5 µg (s.d.; n = 5).

On the basis of a loading of ~220 ± 30 Mg N year−1 and an isotopic signature of ~12.6 ± 7.0‰ for riverine NO3− and a loading of ~390 ± 40 Mg N year−1 and an isotopic signature of ~7.3 ± 1.4‰ for particulate nitrogen, it was estimated that the overall isotopic signature of the total riverine nitrogen input into Western Port was ~9.2 ± 2.8‰ (Eqn 2).
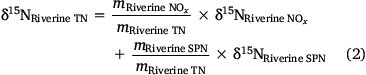
Denitrification
In earlier work by Brandes and Devol (2002), the contribution of denitrification to nitrogen isotope and mass balances was separated into sedimentary- and water column-derived denitrification. However, because the concentration of dissolved inorganic nitrogen in the water column was extremely low (Russell et al. 2016), and no anoxia has ever been recorded in the well mixed water column, it was assumed that water column-derived denitrification represented an insignificant sink, and was ignored. Prior core-incubation experiments by Russell et al. (2016) estimated that the yearly removal of bioavailable nitrogen through denitrification was 228 ± 10 Mg N year−1 across the entirety of Western Port. In the present study, the isotopic value arising from sedimentary denitrification was assumed to be 3.5 ± 2.0‰, on the basis of the global estimates of Brandes and Devol (2002). The globally estimated fractionation factor for sedimentary denitrification used here is similar to those that have been determined on smaller spatial scales, with Alkhatib et al. (2012) calculating a fractionation factor of 4.6 ± 2‰ along the Saint Lawrence Estuary in Canada.
Baywide δ15N values
To determine the average actual isotopic value of sediment nitrogen, against which the model output would be validated, intact sediment samples were collected from sites across Western Port (Fig. 2, Supplementary Table S1) by using Perspex tubes to a depth of ~5 cm. These samples were dried to a constant weight at 60°C for 48 h and then homogenised using a mortar and pestle. Up to 135 mg of the sample was used for analysis of sediment nitrogen content and isotopic analysis, by using the CF-IRMS described above (n = 119; February 2012–November 2016).
![]() |
Marine isotope end-member
Samples of particulate nitrogen were obtained from Cowes and San Remo during the incoming and outgoing tides (Table 2) by using pre-ashed Whatmann 25 mm GF/F paper (n = 43–47; October 2015–January 2017). These filters were processed and analysed using the CF-IRMS, as described previously.
Isotope mass balance to estimate marine import and burial
So as to estimate burial and marine import, the following mass balance was set up:

in which , where msed is the mass of sediment N created in Western Port, moce is mass of N from oceanic sources, mdni is mass denitrified, mriv is riverine-derived N (particulate + dissolved), mfix is N fixation,
and
are atmospheric deposition of NO3− and NH4+. It should be noted that msed, once created, can either be buried or exported from Western Port.
Then, the isotope balance for the sediment in Western Port can be written as follows:

where δ15N is the average nitrogen isotope signature of each compartment and ε is the fractionation factor for denitrification. This equation can be rearranged so that

and msed can be calculated using Eqn 3.
This model assumes Western Port is well mixed on annual timescales which is reasonable given the macrotidal and well mixed nature of the bay.
To propagate the uncertainty in the known parameters onto estimates of moce and msed, we solved Eqn 3 and 4 in R and calculated moce and msed for 5000 independent random draws from a truncated mean ± 1 s.d. of the known parameter values. From the resulting 5000 solutions for moce and msed, a total of 2324 solutions returned a negative and therefore infeasible moce flux; these were removed from the solution set. The results for the combined isotope and mass balance are based on the remaining 2676 solutions.
Data analysis
Differences in isotopic and nitrogen composition of particulate material from Cowes and San Remo were tested for significance using two-factor ANOVA in R (ver. 3.2.2, R Foundation for Statistical Computing, Vienna, Austria). The Tukey’s HSD post hoc test was used in the event that significant responses were returned for the two-factor ANOVA. Data were evaluated graphically by using plots of residuals and boxplots to test for the homogeneity and normality of variances Quinn and Keough (2002). For all analyses, P < 0.05 was chosen as the level of significance for the rejection of the null hypothesis.
Results
Inputs to Western Port were dominated by catchment inputs (612 Mg N year−1), followed by nitrogen fixation (430 Mg N year−1, Table 2). The calculated combined δ15N of the catchment inputs was 9.2‰. Atmospheric deposition was approximately half of nitrogen fixation at 186 Mg N year−1. Both the isotopic signature and the nitrogen content of the particulate nitrogen in the water column showed little variation over the tidal cycle. Samples from Cowes had particulate nitrogen isotopic signatures of between 5.4 and 6.8‰ and a nitrogen concentration of between 50 and 56 µg N L−1 (Fig. 2). Whereas at San Remo, the particulate nitrogen isotopic signatures were between 6.4 and 7.0‰, with a nitrogen concentration of between 29 and 33 µg N L−1 (Fig. 2). On average, it was determined that the isotopic signature of the particulate material was 6.7 ± 1.1‰ across both sites for all sampling periods (Table 2). There was no significant difference in the nitrogen content or δ15N of particulate nitrogen on the incoming and outgoing tides (P > 0.05; two-way ANOVA, with tide and site as factors).
Sediment from various locations around Western Port had an average δ15N of 4.0 ± 1.1‰ (Fig. 3, Table 2). Little variation in the signature was observed around the majority of the bay, with the highest and lowest values being evenly distributed across the north and south of the bay (Supplementary Table S1).
A histogram of the isotope mass balance runs shows that a median oceanic import of 400 Mg N year−1 into Western Port is most likely. The isotope mass balance suggests a most likely burial of 1400 Mg N year−1 (Table 2, Fig. 4).
![]() |
Discussion
Numerous budgets have now been made to examine nitrogen sources in coastal embayments and estuaries (Boynton et al. 1995; McKee et al. 2000; Zeldis and Swaney 2018); however, none has attempted to add additional constraints on these end-members by using nitrogen stable isotopes. This is the first example of a budget of a coastal embayment to use δ15N to constrain different nitrogen sources. Using this approach, we were able to better constrain nitrogen fixation and marine exchange, which are difficult to measure and scale up.
Nitrogen fixation
The mean sediment δ15N of Western Port was 4.0 ± 1.1‰, which is relatively isotopically depleted compared with catchment (9.2 ± 2.8‰) and marine (6.7 ± 1.1‰) sources, suggesting another major source of nitrogen. Although atmospheric sources are also isotopically depleted (δ15N = −1.4 ± 2.9‰), this was a minor source of nitrogen and is well constrained. The most likely source of isotopically depleted nitrogen is nitrogen fixation, for which we have previously measured high rates within the system. Using a combined isotope and mass balance approach, we are able to provide strong additional evidence to support our previous conclusions on the importance of nitrogen fixation within Western Port (Russell et al. 2016).
In many previous studies, the importance of nitrogen fixation to the total inputs of various marine studies has been found to vary from between 3 and 60% (Huber 1986; Howarth et al. 1988; Larsson et al. 2001; Radtke et al. 2012; Korth et al. 2014; Woodland and Cook 2014). The studies that found the highest contribution of nitrogen fixation to the total input of nitrogen typically had cyanobacterial blooms or cyanobacterial mats present. Within Western Port, the contribution of nitrogen fixation to the total input of nitrogen was 28%, placing it in the middle of the range of previous studies; however, they are some of the highest for systems without widespread cyanobacterial blooms and mats. Previous work by Russell et al. (2016) has shown that this nitrogen fixation was occurring as a result of both sulfate-reducing bacteria and ‘freely associated’ cyanobacterial assemblages. We suggest that a key driver of the high rates of nitrogen fixation relative to other inputs of nitrogen are a direct result of relatively oligotrophic conditions that exist within Western Port. It been shown previously that the catchment-derived inputs of nitrogen to Western Port are low on both a local and global scale (Woodland et al. 2015), and the marine waters in this location are also highly nutrient limited (Gibbs et al. 1986), resulting in nitrogen limitation in seagrass meadows found in Western Port (Russell et al. 2016).
Marine and sediment inputs
The isotope mass balance suggested that a median value of 400 Mg N year−1 is imported into Western Port from marine sources. The total N inputs to Western Port from the marine end-members can be estimated on the basis of the daily water exchange of 0.8 km3 (Anon. 2011) and the measured total N concentration of ~50 N μg L−1. This equates a total annual N exchange of 15 000 Mg N year−1 and a retention rate of 3%. We did not measure NOx concentrations in this study; however, the annual average NOx concentration in Bass Strait adjacent to Western Port is ~14 μg L−1 (Gibbs et al. 1986), which equates to an exchange of 4000 Mg N year−1 and a retention rate of 10%. Therefore, the retention rate of N within Western Port from marine sources is relatively low, which is likely a consequence of the low residence time of water (days) in the south of the system where most of water is exchanged (Anon. 2011).
The veracity of the sediment production term in the budget can be assessed by comparison with previously published sedimentation rates (Hancock et al. 2001). These previously reported sedimentation rates range between 2 and 5 mm year−1 with one extreme value of up to 1.6 cm year−1. If we scale this up to the intertidal area of Western Port of ~214 km2 (1/3 of 650 km2), assuming a sediment dry mass of 0.5 kg L−1 and a sediment nitrogen content of 0.32% (based on sediment organic C/6.7 reported in Sharp et al. 2013), then this gives a nitrogen burial rate of 700–1700 Mg N year−1, which is in good agreement with the sediment nitrogen production rate of ~1400 Mg N year−1 estimated from the model.
Conclusions
This study has described the development of a combined mass and isotope balance to constrain the sources of nitrogen to Western Port. The total nitrogen input to Western Port was calculated to be 1400 Mg N year−1, which is remarkably consistent with previous estimates of sedimentation rates within the system. Catchment inputs, nitrogen fixation, marine sources and atmospheric deposition comprised 44, 28, 28 and 13% of nitrogen inputs respectively. Denitrification removed ~16% of total nitrogen inputs.
Data availability
The data used in the mass balance presented here are shown in Table 2 and Supplementary Table S1. The raw data underpinning these data are available from the authors upon request.
Conflicts of interest
The authors declare that they have no conflicts of interest.
Declaration of funding
This work has been funded by the Australian Research Council (LP130100684), Melbourne Water, Parks Victoria and the Victorian Environmental Protection Authority. Douglas Russell was supported by an Australian Government Research Training Program Scholarship (RTP).
Author contributions
P. L. M. Cook, A. J. Kessler, W. W. Wong and D. G. Russell conceived the overall study design. D. G. Russell undertook the sampling and analysis. D. Van Oevelen conceived the mass balance approach implemented here. P. L. M. Cook and D. G. Russell wrote the manuscript with input from all co-authors.
Supplementary material
Supplementary material is available online.
Acknowledgements
The authors thank Andy Longmore and Dr Todd Scicluna for their collection of the sediment and oceanic samples respectively, and Dr Victor Evrard for the initial isotopic analysis of the sediment samples.
References
Alkhatib, M, Lehmann, MF, and Del Giorgio, PA (2012). The nitrogen isotope effect of benthic remineralization–nitrification–denitrification coupling in an estuarine environment. Biogeosciences 9, 1633–1646.| The nitrogen isotope effect of benthic remineralization–nitrification–denitrification coupling in an estuarine environment.Crossref | GoogleScholarGoogle Scholar |
Anon. (2011) Understanding the western port environment: a summary of current knowledge and priorities for future research. Report, Melbourne Water.
Boynton, WR, Garber, JH, Summers, R, and Kemp, WM (1995). Inputs, transformations, and transport of nitrogen and phosphorus in Chesapeake Bay and selected tributaries. Estuaries 18, 285–314.
| Inputs, transformations, and transport of nitrogen and phosphorus in Chesapeake Bay and selected tributaries.Crossref | GoogleScholarGoogle Scholar |
Brandes, JA, and Devol, AH (2002). A global marine‐fixed nitrogen isotopic budget: implications for Holocene nitrogen cycling. Global Biogeochemical Cycles 16, 1120.
| A global marine‐fixed nitrogen isotopic budget: implications for Holocene nitrogen cycling.Crossref | GoogleScholarGoogle Scholar |
Capone, DG, and Kiene, RP (1988). Comparison of microbial dynamics in marine and fresh-water sediments: contrasts in anaerobic carbon catabolism. Limnology and Oceanography 33, 725–749.
| Comparison of microbial dynamics in marine and fresh-water sediments: contrasts in anaerobic carbon catabolism.Crossref | GoogleScholarGoogle Scholar |
Cook, PLM, and Holland, DP (2012). Long term nutrient loads and phytoplankton dynamics in a large temperate Australian lagoon system affected by recurring blooms of Nodularia spumigena. Biogeochemistry 107, 261–274.
| Long term nutrient loads and phytoplankton dynamics in a large temperate Australian lagoon system affected by recurring blooms of Nodularia spumigena.Crossref | GoogleScholarGoogle Scholar |
Cook, P, Evrard, V, and Woodland, RJ (2015). Factors controlling nitrogen fixation in temperate seagrass beds. Marine Ecology Progress Series 525, 41–51.
| Factors controlling nitrogen fixation in temperate seagrass beds.Crossref | GoogleScholarGoogle Scholar |
Eriksson, E (1952). Composition of atmospheric precipitation. Tellus 4, 215–232.
| Composition of atmospheric precipitation.Crossref | GoogleScholarGoogle Scholar |
Eyre, BD, and Mc Kee, LJ (2002). Carbon, nitrogen and phosphorus budgets for a shallow subtropical coastal embayment (Moreton Bay, Australia). Limnology and Oceanography 47, 1043–1055.
| Carbon, nitrogen and phosphorus budgets for a shallow subtropical coastal embayment (Moreton Bay, Australia).Crossref | GoogleScholarGoogle Scholar |
Gibbs, CF, Tomczak Jr, M, and Longmore, AR (1986). The nutrient regime of bass strait. Marine and Freshwater Research 37, 451–466.
| The nutrient regime of bass strait.Crossref | GoogleScholarGoogle Scholar |
Gordon Jr DC, Boudreau BR, Mann KH, Ong J-E, Silvert WL, Smith SV, Wattayakorn G, Wulff F, Yanagi T (1996) ‘LOICZ biogeochemical modelling guidelines.’ (LOICZ)
Hancock G, Olley J, Wallbrink PJ (2001) Sediment transport and accumulation in Western Port. Report, CSIRO Land and Water.
Harmel, RD, Smith, DR, King, KW, and Slade, RM (2009). Estimating storm discharge and water quality data uncertainty: a software tool for monitoring and modeling applications. Environmental Modelling & Software 24, 832–842.
| Estimating storm discharge and water quality data uncertainty: a software tool for monitoring and modeling applications.Crossref | GoogleScholarGoogle Scholar |
Howarth, RW, Marino, R, Lane, J, and Cole, JJ (1988). Nitrogen fixation in freshwater, estuarine, and marine ecosystems. 1. Rates and importance. Limnology and Oceanography 33, 669–687.
| Nitrogen fixation in freshwater, estuarine, and marine ecosystems. 1. Rates and importance.Crossref | GoogleScholarGoogle Scholar |
Huber, AL (1986). Nitrogen fixation by Nodularia spumigena Mertens (Cyanobacteriaceae): 1. Field studies and the contribution of blooms to the nitrogen budget of the Peel–Harvey Estuary, Western Australia. Hydrobiologia 131, 193–203.
| Nitrogen fixation by Nodularia spumigena Mertens (Cyanobacteriaceae): 1. Field studies and the contribution of blooms to the nitrogen budget of the Peel–Harvey Estuary, Western Australia.Crossref | GoogleScholarGoogle Scholar |
Karl D, Michaels A, Bergman B, Capone D, Carpenter E, Letelier R, Lipschultz F, Paerl H, Sigman D, Stal L (2002) Dinitrogen fixation in the world’s oceans. In ‘The nitrogen cycle at regional to global scales’. (Eds EW Boyer, RW Howarth) pp. 47–98. (Springer: Dordrecht, Netherlands)
Korth, F, Deutsch, B, Frey, C, Moros, C, and Voss, M (2014). Nitrate source identification in the baltic sea using its isotopic ratios in combination with a Bayesian isotope mixing model. Biogeosciences 11, 4913–4924.
| Nitrate source identification in the baltic sea using its isotopic ratios in combination with a Bayesian isotope mixing model.Crossref | GoogleScholarGoogle Scholar |
Lansdown KP-M (2009) Biogeochemistry of nitrate in headwater streams and atmospheric deposition of the Dandenong Ranges. Monash University, Melbourne, Vic., Australia.
Larsson, U, Hajdu, S, Walve, J, and Elmgren, R (2001). Baltic Sea nitrogen fixation estimated from the summer increase in upper mixed layer total nitrogen. Limnology and Oceanography 46, 811–820.
| Baltic Sea nitrogen fixation estimated from the summer increase in upper mixed layer total nitrogen.Crossref | GoogleScholarGoogle Scholar |
Liu, K-K, Su, M-J, Hsueh, C-R, and Gong, G-C (1996). The nitrogen isotopic composition of nitrate in the Kuroshio water northeast of Taiwan: evidence for nitrogen fixation as a source of isotopically light nitrate. Marine Chemistry 54, 273–292.
| The nitrogen isotopic composition of nitrate in the Kuroshio water northeast of Taiwan: evidence for nitrogen fixation as a source of isotopically light nitrate.Crossref | GoogleScholarGoogle Scholar |
Mahaffey, C, Williams, RG, Wolff, GA, Mahowald, N, Anderson, W, and Woodward, M (2003). Biogeochemical signatures of nitrogen fixation in the eastern North Atlantic. Geophysical Research Letters 30, 1–4.
| Biogeochemical signatures of nitrogen fixation in the eastern North Atlantic.Crossref | GoogleScholarGoogle Scholar |
McIlvin, MR, and Altabet, MA (2005). Chemical conversion of nitrate and nitrite to nitrous oxide for nitrogen and oxygen isotopic analysis in freshwater and seawater. Analytical Chemistry 77, 5589–5595.
| Chemical conversion of nitrate and nitrite to nitrous oxide for nitrogen and oxygen isotopic analysis in freshwater and seawater.Crossref | GoogleScholarGoogle Scholar | 16131070PubMed |
McKee, LJ, Eyre, BD, and Hossain, S (2000). Transport and retention of nitrogen and phosphorus in the sub-tropical Richmond River Estuary, Australia: a budget approach. Biogeochemistry 50, 241–278.
| Transport and retention of nitrogen and phosphorus in the sub-tropical Richmond River Estuary, Australia: a budget approach.Crossref | GoogleScholarGoogle Scholar |
Mulholland, MR (2007). The fate of nitrogen fixed by diazotrophs in the ocean. Biogeosciences 4, 37–51.
| The fate of nitrogen fixed by diazotrophs in the ocean.Crossref | GoogleScholarGoogle Scholar |
Owens, NJP (1988). Natural variations in 15N in the marine environment. Advances in Marine Biology 24, 389–451.
| Natural variations in 15N in the marine environment.Crossref | GoogleScholarGoogle Scholar |
Proemse, BC, Mayer, B, Fenn, ME, and Ross, CS (2013). A multi-isotope approach for estimating industrial contributions to atmospheric nitrogen deposition in the Athabasca Oil Sands region in Alberta, Canada. Environmental Pollution 182, 80–91.
| A multi-isotope approach for estimating industrial contributions to atmospheric nitrogen deposition in the Athabasca Oil Sands region in Alberta, Canada.Crossref | GoogleScholarGoogle Scholar | 23896680PubMed |
Quinn GP, Keough MJ (2002) ‘Experimental design and data analysis for biologists.’ (Cambridge University Press)
Rabalais, NN, Turner, RE, and Wiseman, WJ (2002). Gulf of Mexico hypoxia, aka 'the dead zone'. Annual Review of Ecology and Systematics 33, 235–263.
| Gulf of Mexico hypoxia, aka 'the dead zone'.Crossref | GoogleScholarGoogle Scholar |
Radtke, H, Neumann, T, Voss, M, and Fennel, W (2012). Modeling pathways of riverine nitrogen and phosphorus in the Baltic Sea. Journal of Geophysical Research: Oceans 117, 1–15.
| Modeling pathways of riverine nitrogen and phosphorus in the Baltic Sea.Crossref | GoogleScholarGoogle Scholar |
Rafter, PA, Bagnell, A, Marconi, D, and DeVries, T (2019). Global trends in marine nitrate n isotopes from observations and a neural network-based climatology. Biogeosciences 16, 2617–2633.
| Global trends in marine nitrate n isotopes from observations and a neural network-based climatology.Crossref | GoogleScholarGoogle Scholar |
Risgaard-Petersen, N, and Jensen, K (1997). Nitrification and denitrification in the rhizosphere of the aquatic macrophyte Lobelia dortmanna L. Limnology and Oceanography 42, 529–537.
| Nitrification and denitrification in the rhizosphere of the aquatic macrophyte Lobelia dortmanna L.Crossref | GoogleScholarGoogle Scholar |
Russell, DG, Warry, FY, and Cook, PLM (2016). The balance between nitrogen fixation and denitrification on vegetated and non-vegetated intertidal sediments. Limnology and Oceanography 61, 2058–2075.
| The balance between nitrogen fixation and denitrification on vegetated and non-vegetated intertidal sediments.Crossref | GoogleScholarGoogle Scholar |
Sharp S, Myers JH, Pettigrove V (2013) An assessment of sediment toxicants in Western Port and major tributaries. Report, University of Melbourne, Vic. Australia.
Steffen, W, Richardson, K, Rockström, J, Cornell, SE, Fetzer, I, Bennett, EM, Biggs, R, Carpenter, SR, de Vries, W, de Wit, CA, Folke, C, Gerten, D, Heinke, J, Mace, GM, Persson, LM, Ramanathan, V, Reyers, B, and Sörlin, S (2015). Planetary boundaries: guiding human development on a changing planet. Science 347, 1259855.
| Planetary boundaries: guiding human development on a changing planet.Crossref | GoogleScholarGoogle Scholar | 25592418PubMed |
Tan K-S, Fox D, Etchells R (2005) ‘Generator for uncertainty measures and load estimates using alternative formulae.’ (Australian Centre for Environmetrics, University of Melbourne: Melbourne, Vic., Australia)
Voss, M, Emeis, KC, Hille, S, Neumann, T, and Dippner, J (2005). Nitrogen cycle of the baltic sea from an isotopic perspective. Global Biogeochemical Cycles 19, GB3001.
| Nitrogen cycle of the baltic sea from an isotopic perspective.Crossref | GoogleScholarGoogle Scholar |
Wong, WW, Grace, MR, Cartwright, I, and Cook, PLM (2014). Sources and fate of nitrate in a groundwater-fed estuary elucidated using stable isotope ratios of nitrogen and oxygen. Limnology and Oceanography 59, 1493–1509.
| Sources and fate of nitrate in a groundwater-fed estuary elucidated using stable isotope ratios of nitrogen and oxygen.Crossref | GoogleScholarGoogle Scholar |
Wong, WW, Pottage, J, Warry, FY, Reich, P, Roberts, K, Grace, MR, and Cook, PLM (2018). Stable isotopes of nitrate reveal different nitrogen processing mechanisms in streams across a land use gradient during wet and dry periods. Biogeosciences 15, 3953–3965.
| Stable isotopes of nitrate reveal different nitrogen processing mechanisms in streams across a land use gradient during wet and dry periods.Crossref | GoogleScholarGoogle Scholar |
Woodland, RJ, and Cook, PLM (2014). Using stable isotope ratios to estimate atmospheric nitrogen fixed by cyanobacteria at the ecosystem scale. Ecological Applications 24, 539–547.
| Using stable isotope ratios to estimate atmospheric nitrogen fixed by cyanobacteria at the ecosystem scale.Crossref | GoogleScholarGoogle Scholar | 24834739PubMed |
Woodland, RJ, Thomson, JR, Mac Nally, R, Reich, P, Evrard, V, Warry, FY, Walker, JP, and Cook, PLM (2015). Nitrogen loads explain primary productivity in estuaries at the ecosystem scale. Limnology and Oceanography 60, 1751–1762.
| Nitrogen loads explain primary productivity in estuaries at the ecosystem scale.Crossref | GoogleScholarGoogle Scholar |
Zeldis, JR, and Swaney, DP (2018). Balance of catchment and offshore nutrient loading and biogeochemical response in four New Zealand coastal systems: implications for resource management. Estuaries and Coasts 41, 2240–2259.
| Balance of catchment and offshore nutrient loading and biogeochemical response in four New Zealand coastal systems: implications for resource management.Crossref | GoogleScholarGoogle Scholar |