Plastid phylogenomics of the Eriostemon group (Rutaceae; Zanthoxyloideae): support for major clades and investigation of a backbone polytomy
Harvey K. Orel


A School of Biosciences, The University of Melbourne, Parkville, Vic. 3010, Australia.
B Royal Botanic Gardens Victoria, Melbourne, Vic. 3004, Australia.
C Queensland Herbarium & Biodiversity Science, Department of Environment & Science, Brisbane Botanic Gardens, Toowong, Qld 4066, Australia.
Australian Systematic Botany 36(5) 355-385 https://doi.org/10.1071/SB23011
Submitted: 28 April 2023 Accepted: 1 August 2023 Published: 22 August 2023
Abstract
Most of Australia’s sclerophyllous Rutaceae belong to a clade informally known as the ‘Eriostemon group’ (including 16 genera, ~209 species). We investigated generic relationships in this group using analyses of complete plastome sequence data for 60 species and analyses of a supermatrix including sequences of four plastome spacer regions for 22 additional species. Maximum likelihood, Bayesian inference, and shortcut coalescent phylogenetic analyses produced congruent phylogenies that were highly supported, except for a series of short unsupported branches in the backbone of the Eriostemon group. We found high support for four major clades branching from this polytomy and discuss evolutionary inferences of generic relationships in each lineage. In an effort to resolve the polytomy, we analysed gene tree topologies in tree space, phylogenetic informativeness with likelihood mapping, and conducted topology tests to assess support for all possible topological resolutions of the polytomy. These approaches did not clarify the polytomy, which may be caused by insufficient data, features of plastome evolution, or rapid radiation. Results from analyses of the combined supermatrix dataset suggest that Philotheca section Philotheca is paraphyletic with regards to Drummondita and Geleznowia. In all phylogenies, Philotheca sections Corynonema and Cyanochlamys were not placed with other members of Philotheca.
Keywords: Asterolasia, Australasia, Australia, Chorilaena, Correa, Crowea, Diplolaena, Drummondita, Eriostemon, Geleznowia, Halfordia, Leionema, likelihood mapping, Muiriantha, Myrtopsis, Nematolepis, Neoschmidia, Phebalium, Philotheca, polytomy, Rutaceae, topology tests, tree space.
Introduction
The Rutaceae is a globally distributed angiosperm family in the order Sapindales that is well-represented in Australia (Orchard 1999). The first major treatments of the family were provided by Engler (1874, 1896, 1931), who placed the Australasian members of the family across four subfamilies (Aurantioideae, Flindersioideae, Rutoideae, and Toddalioideae) and five tribes (Aurantieae, Boronieae, Flindersieae, Toddalieae and Zanthoxyleae). Most Australian taxa were placed in Boronieae, a tribe that Engler (1931) distinguished from the other tribes in subfamily Rutoideae by a broad combination of features pertaining to habit, flower and cotyledon characters, as well as geographic distribution. Subsequent classifications by da Silva et al. (1988) and Hartley (1995, 2003) proposed re-adjustments to the limits of the tribe, but maintained Engler’s (1931) sentiment that the Boronieae predominantly encompasses a species-rich group of sclerophyllous Australian taxa, that chiefly occur outside of rainforests. From morphological analysis, Armstrong (1991) suggested that the tribe was not monophyletic. Several molecular phylogenies published since these works have confirmed that the tribe is polyphyletic (Groppo et al. 2008, 2012; Bayly et al. 2013; Appelhans et al. 2021; Duretto et al. 2023; Joyce et al. 2023). These have established that Boronia Sm., Cyanothamnus Lindl., Neobyrnesia J.A.Armstr. and Zieria Sm. form a clade with members of the Euodia J.R.Forst. & G.Forst. alliance (sensu Kubitzki et al. 2011; e.g. Acronychia J.R.Forst & G.Forst., Brombya F.Muell., Medicosma Hook.f., Melicope J.R.Forst & G.Forst., Perryodendron T.G.Hartley, Picrella Baill., Tetractomia Hook.f.) that is sister to a clade containing most of the genera historically placed in Boronieae (Asterolasia F.Muell., Chorilaena Endl., Correa Andrews, Crowea Sm., Diplolaena R.Br., Drummondita Harv., Eriostemon Sm., Geleznowia Turcz., Leionema (F.Muell.) Paul G.Wilson, Muiriantha C.A.Gardner, Nematolepis Turcz., Phebalium Vent., Philotheca Rudge). Also included in the latter clade are Halfordia F.Muell., Myrtopsis Engl. and Neoschmidia T.G.Hartley, three genera that recent taxonomists have not considered part of tribe Boronieae (Hartley 2001, 2003; Kubitzki et al. 2011). For ease of reference, we hereafter refer to this clade (equivalent to ‘Clade C’ in the work of Bayly et al. 2013) as the Eriostemon group.
The Eriostemon group includes 16 genera and ~209 species that almost all occur naturally in Australia. The exceptions to this are the New Caledonian genera Myrtopsis (~9 spp.) and Neoschmidia (2 spp.), and the New Zealand-endemic Leionema nudum (Hook.) Paul G.Wilson. The group is most diverse in the south-east and south-west of Australia, with only a few species found in the northern half of the continent. Plants in the group occur in various environments, ranging through rainforests, woodlands, savanna, coastal and montane heathlands, semi-arid scrub and desert shrubland. However, most commonly members of the group occur in sclerophyll woodlands and forests that are typically dominated by eucalypts.
The diverse distribution of the group is matched by a large degree of morphological diversity. Plants range in habit from small shrubs to trees (but are mostly small-leaved shrubs); leaf phyllotaxy varies between opposite and alternate arrangement (mostly alternate); inflorescences may be axillary or terminal; flowers are four- or five-merous and solitary or in clusters, cymes or racemes; and fruits range from fleshy drupes to (most commonly) dry, dehiscent follicles.
Because of this morphological diversity and extensive morphological homoplasy (Armstrong 1991), generic classification of the group has been historically difficult. Some genera (e.g. Geleznowia) display a range of highly derived apomorphic characters that support their monophyly but make them hard to place with respect to other genera due to lack of synapomorphies. Others (e.g. Philotheca) are indiscrete in their morphological delimitation and lack strongly defined apomorphies to support their monophyly. This combination of taxa with either highly apomorphic or plesiomorphic morphologies has hindered classification of the whole group.
Several studies have investigated aspects of relationships in the Eriostemon group by using molecular data. These have provided useful resolution of species-level relationships in some genera (e.g. Othman et al. 2010; Bayly et al. 2016; French et al. 2016; Batty et al. 2022), and have established the monophyly of several genera (Mole et al. 2004; Duretto et al. 2023). Despite this body of work, relationships between genera remain poorly understood; the few molecular studies that have sampled the Rutaceae thoroughly have been limited by relatively low taxon sampling from the Eriostemon group or low amounts of sequence data for phylogenetic analysis (e.g. Mole et al. 2004; Bayly et al. 2013; Appelhans et al. 2021; Duretto et al. 2023), leading to poor support for many branches above genus level. Consequently, relationships among genera are unclear and several potential taxonomic issues have been identified but left unresolved.
One major taxonomic issue is the current delimitation of Philotheca, which has been recovered as polyphyletic in several molecular phylogenies but has not been reclassified (Bayly et al. 2013; Appelhans et al. 2021). As currently circumscribed, there are four sections in Philotheca (Wilson 2013a), with 54 accepted, named species and one phrase-named species (Australian Plant Census, see https://biodiversity.org.au/nsl/services/search/taxonomy, accessed April 2023). The type section, Philotheca section Philotheca (34 spp.; eastern and south-western Australia, Fig. 1c), is more closely related to Drummondita (11 spp.; Western Australia, Northern Territory and Queensland, Fig. 1b) and Geleznowia (2 spp.; Western Australia, Fig. 1a) than the three other sections of Philotheca (Bayly et al. 2013; Appelhans et al. 2021). Philotheca section Erionema (F.Muell.) Paul G.Wilson (15 spp.; 14 eastern Australia, one south-western Australia, Fig. 1d), is a monophyletic group that is well-defined by a number of synapomorphies (Wilson 1998a; Bayly 2001; Batty et al. 2022). Philotheca section Corynonema (Paul G.Wilson) Paul G.Wilson (3 spp.; south-eastern and south-western Australia, Fig. 1f) and Philotheca section Cyanochlamys (F.Muell.) Paul G.Wilson (2 spp.; south-western Australia, Fig. 1e) are small sections with diverse floral and vegetative morphologies. The phylogenetic positions of the three non-type sections of Philotheca in the Eriostemon group are yet to be adequately determined.
Distributions of (a) Geleznowia, (b) Drummondita, (c) Philotheca section Philotheca, (d) Philotheca section Erionema, (e) Philotheca section Cyanochlamys, (f) Philotheca section Corynonema. Distributions are also indicated for (b) D. borealis and D. calida (the only species of Drummondita outside of Western Australia, (d) P. brucei (the only species of section Erionema in Western Australia, and (f) the three disjunct species comprising section Corynonema. Maps are based on filtered specimen records from The Australasian Virtual Herbarium (see https://avh.chah.org.au).
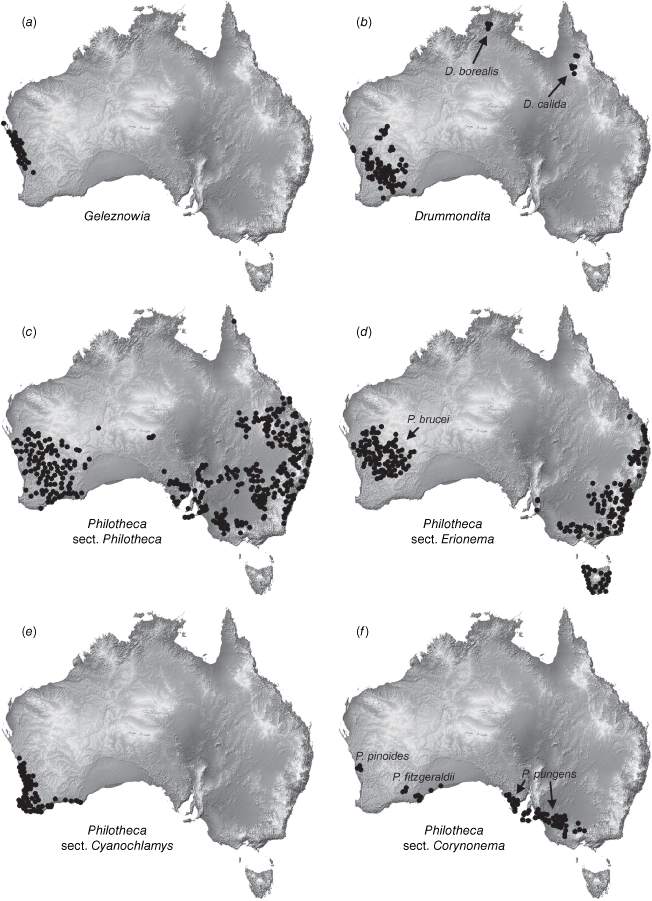
Despite evidence for the polyphyly of Philotheca, relationships among Philotheca section Philotheca, Drummondita and Geleznowia have not yet been resolved well-enough to determine an appropriate taxonomic treatment. In particular, taxonomic changes have not been applied to Philotheca because of uncertainty surrounding the monophyly of section Philotheca; it is not clear whether Geleznowia and Drummondita are sisters to section Philotheca, or nested within it. In the former case, it could be possible to retain these distinctive and long-recognised taxa as separate genera; in the latter case, there would be grounds for including Geleznowia and Drummondita in Philotheca. Morphologically, Drummondita, Geleznowia and Philotheca section Philotheca are mostly distinct in their flowers, which bear differences that are the result of different pollination syndromes (Armstrong 1987). Drummondita has tubular flowers that are often red, orange, yellow or green on the petals, stamens or stigma (Fig. 2b, c), and that are bird pollinated (Armstrong 1987, 1991). The flowers of Geleznowia are bright yellow (to light green) and are unusual for the group in possessing petaloid bracts and sepals (Fig. 2a); the pollinator of these flowers is unknown (Armstrong 1979; Broadhurst and Tan 2001). Species in Philotheca section Philotheca possess comparatively unspecialised flowers that are most commonly white and open-petalled (Fig. 2d, e), although some are pendulous, tubular and more strongly coloured (Fig. 2f–h), and are pollinated variously by beetles, flies, bees, moths, butterflies, and birds (Armstrong 1979). The great degree of variance in derived morphological characteristics (i.e. highly specialised in Drummondita and Geleznowia v. largely unspecialised in section Philotheca) among Philotheca section Philotheca, Drummondita and Geleznowia means that classifying these taxa on the basis of morphology alone is unsatisfactory, owing to the fact that it is difficult to tell whether section Philotheca simply constitutes a group that is defined by symplesiomorphic characteristics. Because of this, further molecular phylogenetic investigation is necessary to clarify whether section Philotheca is monophyletic.
Flowers of Drummondita, Geleznowia and Philotheca section Philotheca: (a) Geleznowia verrucosa, (b) Drummondita hassellii, (c) D. longifolia, (d) Philotheca basistyla, (e) P. difformis subsp. smithiana, (f) P. tubiflora, (g) P. coccinea, and (h) P. salsolifolia. Photographs: Michael Bayly.
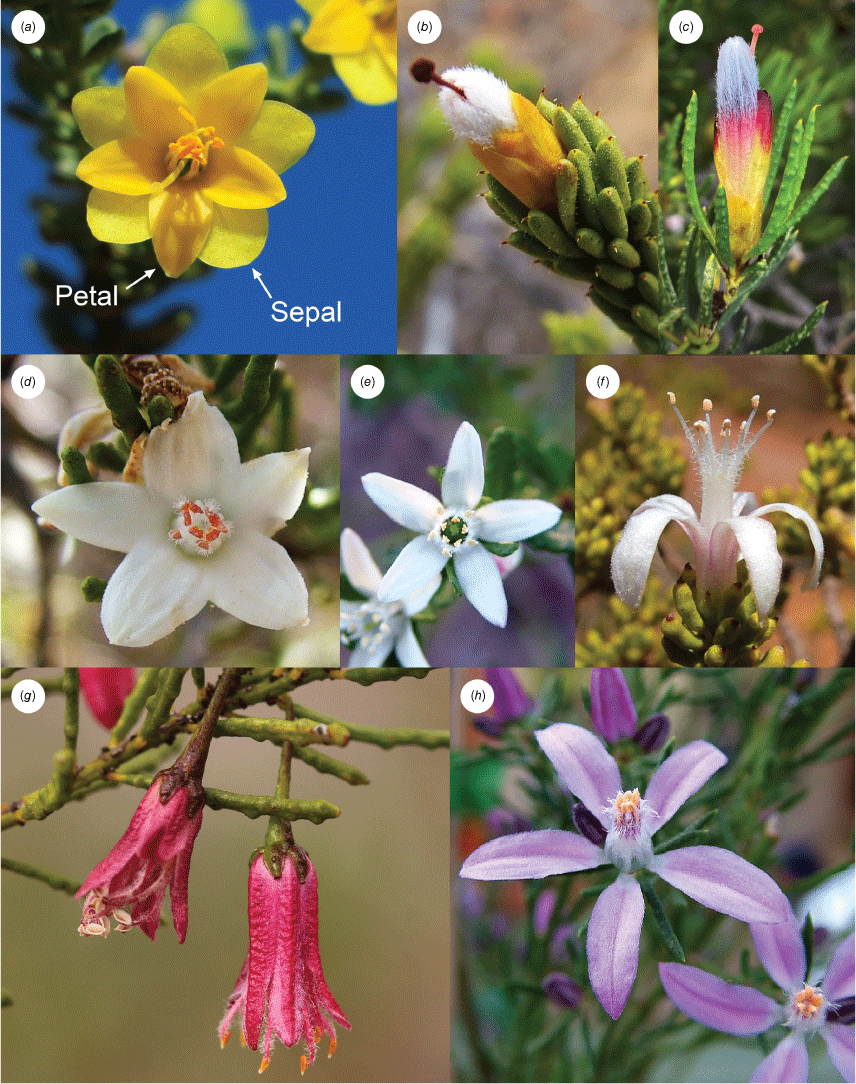
Here, we seek to investigate phylogenetic relationships in the Eriostemon group by using a combination of genome-skimming sequence data and Sanger sequence data. In focussing on this group of taxa, our work expands on that of Mole et al. (2004), Bayly et al. (2013) and Duretto et al. (2023), with the aims of clarifying evolutionary relationships among genera, and testing the monophyly of genera, with particular focus on the genus Philotheca. Our approach represents a notable increase in the amount of taxon sampling and sequence data used to explore phylogenetic relationships in the group, which has previously been studied using only a low number of molecular markers.
Materials and methods
Taxon sampling and marker selection
To investigate relationships in the Eriostemon group, we generated complete plastome sequences for 67 accessions. We aimed to include multiple species from each Australian genus in the group. Two small Australian genera, Geleznowia (two species) and Muiriantha (one species), were each represented by collections of a single species. The New Caledonian genera Neoschmidia and Myrtopsis were also represented by single collections. For outgroups, we sampled members of the clade resolved as sister to the Eriostemon group by previous studies (Groppo et al. 2008; Bayly et al. 2013; Appelhans et al. 2021). This included species from the genera Acronychia, Boronia, Brombya, Cyanothamnus, Euodia, Medicosma, Melicope, Neobyrnesia, Picrella and Zieria. We included plastome sequence data for Zanthoxylum simulans Hance from Hou et al. (2018; GenBank accession number: NC037482) as a more distant outgroup, and with the addition of this taxon, our sampling of whole plastomes totalled 68 accessions (Table 1).
Taxon name | Collector number | Herbarium voucher number(s) | GenBank number (full plastome) | GenBank numbers (Sanger) | Latitude | Longitude | Collection locality | |
---|---|---|---|---|---|---|---|---|
Acronychia laevis | P.I.Forster 33410 | BRI AQ0752244 | OL591157 | – | −25.93 | 152.1 | Australia: Queensland, Grongah National Park. | |
Asterolasia asteriscophora | M.J.Bayly 2564 | MELUD114862a | OL591158 | – | −37.58 | 145.49 | Australia: Victoria, Healesville. | |
Asterolasia drummondii | B.J.Mole 330 | NSW 1003537 | OL591159 | – | −30.8 | 115.6 | Australia: New South Wales. | |
Boronia edwardsii | M.J.Bayly 1974 | MEL 2383596A | OL591160 | – | −35.6 | 138.4 | Australia: South Australia, Yankalilla. | |
Boronia imlayensis | M.J.Bayly 2005 | MELUD105861a | OL591161 | – | – | – | Australia: Cultivated, Australian National Botanic Garden (loc. 23a, Prop ID 749585). | |
Boronia ternata | M.J.Bayly 1931 | MEL 2383603A | OL591162 | – | −31.26 | 120.05 | Australia: Western Australia, Boorabbin National Park. | |
Brombya platynema | P.I.Forster 34088 | HO550407 | OL591163 | – | −17.58 | 145.7 | Australia: Queensland, Wooroonooran National Park. | |
Chorilaena anceps | B.J.Mole 475 | NSW 1003674 | OL591221 | – | −35.01 | 117.92 | Australia: Western Australia, Albany. | |
Chorilaena euphemiae | B.J.Mole 424 | NSW 1003630 | OL591222 | – | −33.99 | 122.14 | Australia: Western Australia, Mount Le Grand. | |
Chorilaena quercifolia | M.J.Bayly 1954 | MEL 2383575A | OL591164 | – | −34.1 | 115.98 | Australia: Western Australia, Netic State Forest. | |
Correa alba | M.J.Bayly 1876 | MELUD105867a | OL591165 | – | −38.4 | 144.18 | Australia: Victoria, Anglesea. | |
Correa glabra | M.J.Bayly 2476 | MELUD127019a | OL591166 | – | −36.08 | 143.23 | Australia: Victoria, Mount Wycheproof. | |
Correa lawrenceana var. grampiana | M.J.Bayly 1988 | MEL 2383594A | OL591167 | – | −37.29 | 142.59 | Australia: Victoria, Grampians. | |
Correa lawrenceana var. latrobeana | M.J.Bayly 2567 | MELUD114861a | OL591168 | – | −37.53 | 145.51 | Australia: Victoria, Toolangi. | |
Crowea angustifolia var. platyphylla | M.J.Bayly 1953 | MEL 2383618A | OL591169 | – | −34.58 | 116.4 | Australia: Western Australia, Shannon National Park. | |
Crowea exalata subsp. exalata | D.J.Ohlsen s.n. | MELUD121723a | OL591170 | – | −37.37 | 148.22 | Australia: Victoria, W Tree. | |
Crowea exalata var. revoluta | M.J.Bayly 1992 | MELUD105865a | OL591171 | – | −36.67 | 144.25 | Australia: Victoria, Greater Bendigo National Park. | |
Crowea saligna | D.J.Ohlsen s.n. | MELUD121722a | OL591172 | – | −33.73 | 151.24 | Australia: New South Wales, Sydney. | |
Cyanothamnus anemonifolius | M.J.Bayly 2562 | MELUD114859a | OL591173 | – | −37.74 | 144.31 | Australia: Victoria, Brisbane Ranges National Park. | |
Diplolaena drummondii | M.J.Bayly 1956 | MEL 2383571A | OL591174 | – | −33.37 | 115.97 | Australia: Western Australia, Wellington National Park. | |
Diplolaena obovata | M.J.Bayly 1908 | MEL 2383570A | OL591175 | – | −30.05 | 115.07 | Australia: Western Australia. | |
Drummondita calida | P.I.Forster 22556 | BRI AQ0605109 | OL591176 | – | −17.52 | 143.75 | Australia: Queensland Bulleringa National Park. | |
Drummondita fulva | A.S.Markey 6212 | MELUD105908a | OL591177 | – | −29.1 | 116.9 | Australia: Western Australia, Blue Hills Range. | |
Drummondita hassellii | M.J.Bayly 1925 | MEL 2383612A | OL591178 | – | −31.32 | 117.94 | Australia: Western Australia, Trayning. | |
Drummondita hassellii | M.J.Bayly 1928 | MEL 2383568A | – | OL660700; OM744163; OL660675; OL697857 | −31.28 | 119.83 | Australia: Western Australia, Yellowdine Nature Reserve. | |
Eriostemon australasius | P.I.Forster 34192 | BRI AQ0743514 | OL591179 | – | −26.01 | 153.05 | Australia: Queensland, Great Sandy NP. | |
Eriostemon banksii | P.I.Forster 33960 | BRI AQ0743323 | OL591180 | – | −11.71 | 142.86 | Australia: Queensland, Cape York Peninsula. | |
Euodia pubifolia | P.I.Forster 25751 | BRI AQ0607159 | OL591181 | – | −16.14 | 145.43 | Australia: Queensland, Daintree National Park. | |
Geleznowia verrucosa | B.J.Mole 344 | NSW 1003545 | OL591182 | – | −29.99 | 115.91 | Australia: Western Australia, Coorow. | |
Geleznowia verrucosa | M.J.Bayly 1910 | MEL 2383587A | OL591183 | – | −29.8 | 115.49 | Australia: Western Australia, Tathra National Park. | |
Geleznowia verrucosa | M.J.Bayly 1909 | MEL 2383586A | – | OL660701; OM744150; OM803177; OL697858 | −29.8 | 115.49 | Australia: Western Australia, Tathra National Park. | |
Halfordia kendack | D.G.Fell 10829 | CNS 149723.1 | OL591184 | – | −10.18 | 142.23 | Australia: Queensland, Moa Island. | |
Halfordia kendack | G.&N.Sankowksy 3019 | MELUD105887a | OL591188 | – | – | – | Australia: Cultivated Tolga. Arboretum number 276. | |
Halfordia kendack | P.I.Forster 34073 | BRI AQ0743506 | OL591185 | – | −17.41 | 145.7 | Australia: Queensland, Topaz. | |
Halfordia kendack | P.I.Forster 34090 | BRI AQ0743446 | OL591186 | – | −17.45 | 145.48 | Australia: Queensland, Herberton Range Forest Reserve. | |
Halfordia kendack | P.I.Forster 34580 | BRI AQ0745524 | OL591187 | – | −12.25 | 143.09 | Australia: Queensland, Cape York Peninsula. | |
Leionema beckleri | P.I.Forster 33439 | BRI AQ0752479 | OL591189 | – | −28.22 | 153.21 | Australia: Queensland, Lamington National Park. | |
Leionema ellipticum | P.I.Forster 25021 | BRI AQ0606667 | OL591190 | – | −15.82 | 145.28 | Australia: Queensland, Cedar Bay National Park. | |
Leionema lamprophyllum subsp. obovatum | M.J.Bayly 2563 | MELUD114858a | OL591191 | – | −37.74 | 144.31 | Australia: Victoria, Brisbane Ranges National Park. | |
Leionema rotundifolium | P.I.Forster 34469 | BRI AQ0745286 | OL591192 | – | −28.83 | 151.96 | Australia: Queensland, Girraween National Park. | |
Medicosma cunninghamii | P.I.Forster 33501 | BRI AQ0752476 | OL591193 | – | −26.45 | 152.97 | Australia: Queensland, Sunshine Coast. | |
Melicope hayesii | P.I.Forster 36183 | BRI AQ0813873 | OL591194 | – | −28.26 | 153.16 | Australia: Queensland, Lamington National Park. | |
Muiriantha hassellii | B.J.Mole 474 | NSW 1003673 | OL591196 | – | −34.4 | 118 | Australia: Western Australia, Plantagenet. | |
Myrtopsis sp. | J.Munzinger 3458 | NOU014250; MNHN-P-P04759709 | OL591197 | – | −22.1 | 166.64 | New Caledonia: Riviére Bleu. | |
Nematolepis phebalioides | A.S.Markey 6215 | MEL 2337320A; PERTH 8114846 | OL591198 | – | −33.66 | 120.28 | Australia: Western Australia, Ravensthorpe Range. | |
Nematolepis squamea | P.I.Forster 34811 | BRI AQ745513 | OL591199 | – | – | – | Australia: New South Wales, Wooyung, near Billinudgel. | |
Nematolepis wilsonii | M.J.Bayly 2568 | MELUD114864a | OL591200 | – | −37.83 | 144.98 | Australia: Cultivated Royal Botanic Gardens Victoria. | |
Neobyrnesia suberosa | M.J.Bayly 1904 | MEL 2383567A | OL591201 | – | −12.44 | 132.97 | Australia: Northern Territory, Kakadu National Park. | |
Neoschmidia pallida | P.H.Weston 3303 | NSW783008 | OL591202 | – | – | – | New Caledonia: Cultivated Royal Botanic Garden Sydney, ex. Mount Dore. | |
Phebalium clavatum | B.J.Mole 398 | NSW 1003609 | OL591203 | – | −31.2 | 121.3 | Australia: Western Australia, Coolgardie. | |
Phebalium elegans | B.J.Mole 403 | NSW 1003615 | OL591204 | – | −32.06 | 122.72 | Australia: Western Australia, east of Norseman. | |
Phebalium longifolium | P.I.Forster 25088 | BRI AQ0678653 | OL591205 | – | −17.32 | 145.42 | Australia: Queensland, Mount Baldy State Forest. | |
Phebalium multiflorum | R.Butcher 1280 | MELUD105904A; PERTH 8143110 | OL591195 | – | −33.66 | 120.27 | Australia: Western Australia, Ravensthorpe Range. | |
Phebalium stenophyllum | M.J.Bayly 2560 | MELUD127020a | OL591206 | – | −36.61 | 141.75 | Australia: Victoria, Little Desert. | |
Phebalium tuberculosum | B.J.Mole 375 | NSW 1003583 | OL591207 | – | −31.48 | 118.33 | Australia: Western Australia, east of Merridin. | |
Phebalium whitei | P.I.Forster 34467 | BRI AQ0745285 | OL591208 | – | −28.83 | 151.96 | Australia: Queensland, Girraween National Park. | |
Philotheca acrolopha | W.W.Cooper 2048 | BRI AQ0745516; CNS 134918.1 | – | OL660702; OM744164; OL660676; OL697859 | −12.75 | 143.21 | Australia: Queensland, Mount Tozer, Iron Range. | |
Philotheca angustifolia subsp. angustifolia | M.J.Bayly 1990 | MEL 2383589A | OL591209 | – | −36.55 | 144.35 | Australia: Victoria, Greater Bendigo National Park. | |
Philotheca angustifolia subsp. montana | M.J.Bayly 1871 | MELUD105857a | – | OL660703; OM744155; OL660677; OL697860 | −36.88 | 142.37 | Australia: Victoria, Mount Zero, northern Grampians. | |
Philotheca apiculata | M.J.Bayly 1939 | MELUD105856a | – | OL660704; OM744147; OL660678; OL697861 | −32.2 | 121.8 | Australia: Western Australia. | |
Philotheca basistyla | M.J.Bayly 1924 | PERTH 7810989 | – | OL660705; OM744165; OL660679; OL697862 | −31.3 | 118 | Australia: Western Australia. | |
Philotheca brevifolia | M.J.Bayly 322 | PERTH 7421087 | – | OL660706; OM744167; OL660680; OL697863 | −33.97 | 146.17 | Australia: New South Wales, Cocoparra Nature Reserve. | |
Philotheca ciliata | P.I.Forster 29594 | BRI AQ0647786; HO538812; NE 86281 | – | OL660707; OM744168; OL660681; OL697864 | −28.39 | 151.27 | Australia: Queensland, Biggs Road, 18 km east of Inglewood. | |
Philotheca coateana | M.J.Bayly 1936 | MELUD105855a | – | OL660708; OM744156; OL660682; OL697865 | −29.2 | 120.1 | Australia: Western Australia. | |
Philotheca coccinea | M.J.Bayly 1929 | MEL 2383614A | – | OL660709; OM744149; OL660683; OL697866 | −31.27 | 120.02 | Australia: Western Australia, Boorabbin National Park. | |
Philotheca cuticularis | P.I.Forster 35315 | BRI AQ0813977; MEL 2340118A | – | OL660710; OM744145; OL660684; OL697867 | −25.71 | 144.46 | Australia: Queensland, near Little Hell Hole Waterhole, Milo Station. | |
Philotheca deserti subsp. deserti | M.J.Bayly 1919 | MEL 2383584A | – | OL660711; OM744144; OL660685; OL697868 | −29.78 | 117.03 | Australia: Western Australia, Great Northern Highway, 93.8 km south-west of Paynes Find. | |
Philotheca difformis subsp. difformis | M.T.Mathieson 274 | MEL 2339553A | – | OL660712; OM744159; OL660686; OL697869 | −26.11 | 147.64 | Australia: Queensland, western end of ‘Currawarra’. | |
Philotheca difformis subsp. smithiana | M.J.Bayly s.n. | MELUD121708a | OL591210 | – | −26.41 | 152.98 | Australia: Queensland, Tinbeerwah. | |
Philotheca ericifolia | M.J.Bayly 203 | MEL 2278545A | – | OL660713; OM744161; OL660687; OL697870 | −30.61 | 149.32 | Australia: New South Wales, Pilliga East State Forest. | |
Philotheca fitzgeraldii | M.J.Bayly 1942 | MEL 2383574A | OL591211 | – | −32.63 | 121.55 | Australia: Western Australia. | |
Philotheca gardneri | M.J.Bayly 1949 | MEL 2383569A | OL591212 | – | −33.46 | 119.99 | Australia: Western Australia, Ravensthorpe. | |
Philotheca glabra | M.J.Bayly 1917 | MEL 2383617A | – | OL660714; OM744146; OL660688; OL697871 | −29.59 | 117.15 | Australia: Western Australia, Great Northern Highway, 1 km south of White Wells turn-off. | |
Philotheca linearis | J.J.Bruhl 2864 | NE 113630 | – | OL660715; OM744157; OL660689; OL697872 | – | – | – | |
Philotheca linearis | M.J.Bayly 186 | MELUD105844a | – | OL660716; OM744158; OL660690; OL697873 | −31.05 | 145.24 | Australia: New South Wales, Beside Gidgee Road, 17.7 km west of Louth-Cobar Road. | |
Philotheca myoporoides subsp. myoporoides | M.J.Bayly 2565 | MELUD114860a | OL591213 | – | −37.53 | 145.52 | Australia: Victoria, Toolangi. | |
Philotheca nodiflora subsp. lasiocalyx | M.J.Bayly 1962 | MELUD105840a | OL591214 | – | – | – | Australia: Cultivated ex. Kuranga. | |
Philotheca pachyphylla | M.J.Bayly 1932 | MEL 2383619A | – | OL660717; OM744153; OL660691; OL697874 | −31.04 | 120.84 | Australia: Western Australia, 3.8 km west of Bullabulling on Great Eastern Highway. | |
Philotheca pinoides | M.J.Bayly 11 | MELUD105845a | OL591215 | – | −29.8 | 115.47 | Australia: Western Australia, east of Eneabba. | |
Philotheca pungens | M.J.Bayly 1872 | MELUD105849a | OL591216 | – | −36.92 | 142.43 | Australia: Victoria, Grampians. | |
Philotheca rhomboidea | M.J.Bayly 1950 | MEL 2383576A | – | OL660718; OM744154; OL660692; OL697875 | −33.36 | 119.87 | Australia: Western Australia, Lake King-Ravensthorpe Road, near Lake Chidnup. | |
Philotheca salsolifolia subsp. salsolifolia | M.J.Bayly 1961 | MELUD105841a | – | OL660719; OM744169; OL660693; OL697876 | – | – | Australia: Victoria, Cultivated in Rosanna. | |
Philotheca sericea | M.J.Bayly 1916 | MEL 2383579A | – | OL660720; OM744151; OL660694; OL697877 | −29.29 | 117.48 | Australia: Western Australia, ~20 km west-south-west of Paynes Find. | |
Philotheca spicata | M.J.Bayly 1907 | MEL 2383588A | OL591217 | – | −30.07 | 115.53 | Australia: Western Australia. | |
Philotheca sporadica | M.T.Mathieson 217 | MEL 2339573A | – | OL660721; OM744160; OL660695; OL697878 | −27.07 | 150.84 | Australia: Queensland, Condamine Highway, east of Kogan. | |
Philotheca thryptomenoides | M.J.Bayly 1921 | MEL 2383582A | – | OL660722; OM744162; OL660696; OL697879 | −29.78 | 117.03 | Australia: Western Australia, Great Northern Highway 93.8 km south-west of Paynes Find. | |
Philotheca tomentella | M.J.Bayly 1913 | MEL 2383616A | OL591218 | – | −28.44 | 116.04 | Australia: Western Australia, Pindar. | |
Philotheca trachyphylla | M.J.Bayly 1900 | MELUD105850a | OL591219 | – | −37.73 | 148.09 | Australia: Victoria, Nowa Nowa. | |
Philotheca tubiflora | M.J.Bayly 1934 | MELUD105854a | – | OL660723; OM744152; OL660697; OL697880 | −28.3 | 122.6 | Australia: Western Australia. | |
Philotheca verrucosa | M.J.Bayly 2199 | MELUD121711a | – | OL660724; OM744166; OL660698; OL697881 | −37.89 | 144.22 | Australia: Victoria, Brisbane Ranges. | |
Philotheca virgata | M.J.Bayly 266 | MELUD105843a | – | OL660725; OM744148; OL660699; OL697882 | −37.4 | 149.26 | Australia: Victoria, Coopracambra National Park, Mount Kaye walking track. | |
Picrella glandulosa | M.J.Bayly 2104 | MEL 2383678A | OL591220 | – | −20.32 | 164.42 | New Caledonia: Province Nord. | |
Zanthoxylum simulans | see Hou et al. (2018) | NC037482 | ||||||
Zieria arborescens subsp. arborescens | M.J.Bayly 2566 | MELUD114863a | OL591223 | – | −37.53 | 145.51 | Australia: Victoria, Toolangi. |
Genbank numbers for Sanger sequences are listed in the order: psbA–trnH; rpl32–trnL; trnL–trnF; trnQ–rps16. Samples included from previous existing sequences on GenBank are in bold.
For a more detailed evaluation of relationships among Philotheca, Drummondita and Geleznowia, we generated Sanger sequence data for four plastome intergenic spacers (psbA–trnH, rpl32–trnL, trnL–trnF, trnQ–rps16) from an additional 22 species in this alliance (Table 1). These markers have been successfully used in other phylogenetic studies focussed on the Australian Rutaceae (e.g. Neal et al. 2019; Duretto et al. 2020). Information on the taxonomic coverage of our sampling across the Eriostemon group is provided in Table 2.
Genus | Section | Number of species sampled (full plastome data) | Number of species sampled (Sanger data) | Total number of species | |
---|---|---|---|---|---|
Asterolasia | 2 | 19 | |||
Chorilaena | 3 | 4 | |||
Correa | 3 | 11 | |||
Crowea | 3 | 3 | |||
Diplolaena | 2 | 15 | |||
Drummondita | 3 | (1) | 11 | ||
Eriostemon | 2 | 2 | |||
Geleznowia | 1 | (1) | 2 | ||
Halfordia | 1 | 1–3 | |||
Leionema | 4 | 28 | |||
Muiriantha | 1 | 1 | |||
Myrtopsis | 1 | ~9 | |||
Nematolepis | 3 | 7 | |||
Neoschmidia | 1 | 2 | |||
Phebalium | 7 | 38 | |||
Philotheca | Corynonema | 3 | 3 | ||
Cyanochlamys | 2 | 2 | |||
Erionema | 2 | 2 | 15 | ||
Philotheca | 4 | 20 (2) | 34 | ||
Total | 48 | 22 (4) | ~206–209 |
Numbers in parentheses indicate where the same species was sampled in both the full plastome and Sanger datasets. Total number of species includes described taxa only (i.e. phrase-named species are excluded).
DNA extractions, library preparation and sequencing
DNA extractions for shotgun sequencing were performed following a modified CTAB protocol based on Shepherd and McLay (2011) by using ~25 mg of leaf tissue. DNA libraries for these samples were prepared ‘in-house’ following the protocol of Schuster et al. (2018). Libraries were sequenced on Illumina HiSeq 2000 (HiSeq SBS Kit, 2 × 125-bp paired-end reads) and Illumina NextSeq 550 (Mid-output kit, 2 × 150-bp paired-end reads) sequencing platforms at AgriBio, Centre for AgriBioscience (LaTrobe University) and the Walter and Eliza Hall Institute of Medical Research (WEHI) in Melbourne, Vic., Australia.
DNA extractions for Sanger sequencing from the additional samples of the Philotheca section Philotheca–Drummondita–Geleznowia alliance were performed on 25 mg of leaf tissue by using a DNeasy Plant Mini Kit (Qiagen), following the manufacturer’s protocol, with a final elution volume of 100 μL. The rpl32–trnL, trnL–trnF and trnQ–rps16 regions were amplified from 10 to 20 ng of genomic DNA by following the method of Neal et al. (2019). The psbA–trnH region was amplified with psbAF (Sang et al. 1997) and trnH2 (Tate and Simpson 2003) primers, following the method of Duretto et al. (2020). Polymerase chain-reaction (PCR) products were purified following Neal et al. (2019) and sequenced at the Australian Genome Research Facility (AGRF) in Melbourne, Vic., Australia.
Sequence assembly, editing and alignment
Raw Illumina reads were de novo assembled into contigs with CLC Genomics Workbench (ver. 9.5.1, Qiagen) by using default settings. Contigs and raw reads were imported into Geneious Prime (ver. 2021.1.1, see http://www.geneious.com) and plastome sequences were assembled in this software. Initially, the plastome sequence of Acronychia laevis J.R.Forst. & G.Forst. was built using the plastome sequence of Zanthoxylum schinifolium Siebold & Zucc. (GenBank: NC030702) as a reference. To assemble full plastome sequences we adopted an approach of using a closely related, already-assembled plastome as the reference for the next assembly. For example, the assembled Acronychia laevis plastome sequence was used as a reference for the assembly of other closely related species (e.g. Boronia imlayensis P.H.Weston & Duretto, Medicosma cunninghamii (Hook.) Benth. & Hook.f., Melicope hayesii T.G.Hartley). Where they were more closely related to unassembled samples, these assemblies were then used as references for other plastome assemblies (e.g. Boronia imlayensis as the reference for B. edwardsii Benth. and B. ternata Endl.), and so on. Our assembly pipeline involved first mapping contigs to the selected reference by using custom sensitivity settings with three iterations, gaps allowed, Maximum Per Read set to 40% and Maximum Gap Size set to 1000. The resulting consensus sequence was exported, with any sites between contigs (i.e. with no contig coverage) called as the reference sequence. To replace reference sites and verify the draft sequence, the paired-end reads for the same sample were mapped to the consensus with three to five iterations, gaps allowed Maximum Per Read set to 40% and Maximum Gap Size set to 40. Reads mapped to the consensus were visually inspected for any erroneous mapping, with errors (such as poorly mapped reads at regions with mononucleotide repeats) being corrected manually. We then exported the read-mapped consensus using a site consensus threshold of 75% (i.e. sites with <75% consensus were treated as ambiguities) and sites with coverage of less than three reads were treated as N values (unidentified nucleotides). The plastome sequence of Neoschmidia pallida T.G.Hartley was found to possess significant structural rearrangements and differed substantially from any other plastome sequences in our dataset. Because of this, we used the ‘get_organelle_from_reads.py’ script of GetOrganelle (ver. 1.7.5.3, see https://github.com/Kinggerm/GetOrganelle; Jin et al. 2020) to assemble a draft plastome of that accession. This draft plastome was then treated in the same manner as were the other initial exported consensuses (following the contig-mapping step) to produce a final plastome sequence. Across all samples, the mean number of reads mapped to the final plastome sequence of each sample was 148 418, with an average read depth of 140 reads per sample (individual statistics provided in Supplementary Table S1). Owing to frequent shifting of gene boundaries across taxa preventing the accurate transfer of annotations between plastome sequences, we batched annotated plastomes by using GeSeq (see https://chlorobox.mpimp-golm.mpg.de/geseq.html; Tillich et al. 2017) with default annotators. Annotations were then manually checked, corrected and Ns were annotated as GenBank features by using a custom Python script.
Following assembly, we opted to split plastome sequences into individual loci, rather than align whole plastome sequences for increased alignment accuracy. For this process, we used a workflow that employed several steps in Geneious to extract protein-coding sequences (CDS) and non-coding spacer loci. tRNA and rRNA sequences were excluded because of the often short length and low variability of these loci. For CDS loci, introns were removed (~13 000 bp per sample) and exons concatenated. Non-coding spacer loci were extracted, aligned, and sites with more than 30% gaps were removed to prevent the inclusion of non-homologous sites, and loci were then extracted from the alignment file to individual sequence files. For loci present in both inverted repeat regions of the plastome, the copy from inverted repeat B was removed. In total, 41 loci with fewer than 62 sequences were removed, and the remaining 183 CDS and spacer loci were aligned separately using MAFFT (ver. 7.450, see https://mafft.cbrc.jp/alignment/software/; Katoh et al. 2002; Katoh and Standley 2013) with default settings. Individual locus alignments were manually checked for errors and two alignments were removed owing to poor quality (the ndhA–ndhH spacer, being an alignment of a single base; the rps3–rpl22 spacer, with sequences of varying lengths owing to this locus traversing an inverted repeat boundary in some taxa). The alignments were then concatenated and, to reduce the potential for inclusion of non-homologous sites, sites with more than 80% gaps were removed to produce a final ‘phylogenomic’ alignment of the 68 samples that was 106 885 base pairs in length (65 778 bp CDS, 41 107 bp non-coding).
In addition to the plastome assemblies, we also assembled the complete 18S–5.8S–26S nuclear ribosomal cistron from the genome-skimming data. These sequences were intended to be analysed to test for cytonuclear discordance and assess genetic relationships, but the resulting phylogeny was poorly supported overall and results are largely not discussed here owing to the equivocality of most branches in the tree. The methods and results of analysis of the nuclear ribosomal cistron sequences are presented in the ‘Methods and results of analyses of 18S–5.8S–26S nuclear ribosomal cistron sequences from shotgun-sequenced samples’ section of the Supplementary material (hereafter the Supplementary ‘Methods and results’ section).
Several studies have shown that phylogenetic resolution of Sanger sequence datasets can be improved by integrating them with phylogenomic datasets (e.g. Bardon et al. 2016; Williams et al. 2016; Hammer et al. 2019). The integration of these different types of data can be undertaken either at the alignment stage, with Sanger data sequences appended to large-scale alignments to form a ‘supermatrix’, or by analysing the datasets separately and running phylogenetic analyses of Sanger data under a constrained topology resolved by the genomic dataset. Preliminary tests of both methods on our data found that the supermatrix approach produced a better-resolved and more highly supported tree than did the constrained topology approach, a result also found by Williams et al. (2016). Hence, we opted to use the supermatrix approach to investigate relationships in Philotheca, Drummondita and Geleznowia. Sanger sequences were edited in Sequencher (ver. 4.10.1, Gene Codes Corporation) and imported into Geneious. Sanger sequence regions psbA–trnH, rpl32–trnL, trnL–trnF and trnQ–rps16 were aligned with the same spacer regions as taken from the complete plastomes, and sites with more than 30% gaps were removed to account for potentially non-homologous alignment sites. The four integrated locus alignments were concatenated with the remaining plastome-derived locus alignments to produce a final ‘supermatrix’ alignment of 94 samples that was 106 714 base pairs in length. Complete plastome and Sanger sequences were deposited in GenBank (Table 1).
Phylogenetic analyses
We analysed the phylogenomic alignment by using maximum likelihood and Bayesian inference approaches in IQ-TREE (ver. 2.1.3, see http://www.iqtree.org/; Minh et al. 2020b) and MrBayes (ver. 3.2.7a, see https://nbisweden.github.io/MrBayes/download.html; Ronquist et al. 2012) under three partition schemes: (1) unpartitioned, (2) coding (CDS) v. non-coding sequences and (3) partitioned by codon position and coding v. non-coding sequences. We also ran an IQ-TREE analysis, with the data partitioned by locus. To check for possible site saturation, we translated the CDS locus alignments to amino acids and analysed them with IQ-TREE, comparing the resulting phylogeny with those produced from DNA alignments. To estimate branch support across our IQ-TREE analyses, we employed default ultrafast bootstrap (UFBoot) (Hoang et al. 2018) and SH-aLRT (Guindon et al. 2010), with each being set to 1000 replicates. For these analyses, best-fit models were estimated in IQ-TREE using ModelFinder (see http://www.iqtree.org/ModelFinder/; Kalyaanamoorthy et al. 2017). For the Bayesian analyses, the ‘-mset’ option was used to restrict model searches to those implemented in MrBayes. Information on partitioning and model selection is provided in Supplementary Table S2. MrBayes analyses were run for 1 000 000 generations with a 25% burn-in; we manually checked that the standard deviation of split frequencies was below 0.01 by the end of each run. Following this, we assessed convergence in Tracer (ver. 1.7.2, see http://beast.community/tracer; Rambaut et al. 2018) to ensure that effective sample sizes were above 200 for all parameters. To evaluate our data further, we produced gene trees for all loci with IQ-TREE and used these as input for coalescent analysis in ASTRAL-III (ver. 5.7.7, see https://github.com/smirarab/ASTRAL; Zhang et al. 2018), inferring local posterior probability as support values. We also used the option ‘−t 10’ to test for possible hard polytomies in the species tree (Sayyari and Mirarab 2018). In addition, we used the individual locus alignments and gene trees to calculate concordance factors for the phylogeny produced under the unpartitioned scheme in IQ-TREE, following the method of Minh et al. (2020a); this approach calculates site concordance (sCF: the fraction of decisive alignment sites supporting a branch in the species tree) and gene concordance (gCF: the proportion of gene trees that are concordant with any particular branch in the species tree).
On the basis of results from analyses of the phylogenomic alignment (in which partitioning did not affect topology – see Results), we analysed the supermatrix alignment with IQ-TREE and MrBayes following the same approach as used for analyses of the phylogenomic alignment, with data treated as unpartitioned.
Investigating a polytomy in the backbone of the Eriostemon group
The phylogenetic analyses described above all produced phylogenetic trees with a large polytomy along the backbone of the Eriostemon group. To further investigate this polytomy, we performed additional analyses with the aim of assessing potential causes of the polytomy and increasing phylogenetic resolution.
Previous studies have found that analysis of phylogenetic tree space can improve phylogenetic resolution and low branch support by accounting for topological discordance among loci (Duchêne et al. 2018; Foster et al. 2018). To investigate topological congruence across gene trees in the full-plastome dataset we followed the methods of Duchêne et al. (2018) and Foster et al. (2018), with some modifications. Loci with incomplete sampling (i.e. n < 68) were removed to avoid topological differences owing to missing tips, for a total of 148 input loci (71 CDS, 77 non-coding spacers; further details are provided in Supplementary Table S3). Gene trees were estimated using maximum likelihood in RAxML (ver. 8.2.11, see https://github.com/stamatak/standard-RAxML; Stamatakis 2014), assuming a GTR + GAMMA substitution model with rapid bootstrapping and 100 replicates. We used the R package treespace (ver. 1.1.4.1, see https://cran.r-project.org/package=treespace; Jombart et al. 2017) to calculate unweighted Robinson–Foulds pairwise distances (Penny and Hendy 1985) between all pairs of trees generated with RAxML. We used the R package CLUSTER (ver. 2.1.4, M. Maechler, P. Rousseeuw, A. Struyf, M. Hubert and K. Hornik, see https://CRAN.R-project.org/package=cluster) to calculate the optimal number of clusters (k) by using the gap statistic (Tibshirani et al. 2001) for kmax = 16. Gene trees were separated on the basis of this optimal cluster number, and phylogenetic trees for each cluster were generated in ASTRAL. To further assess the topology of each cluster, the individual locus alignments were separated on the basis of the optimal cluster number, concatenated, and analysed in IQ-TREE.
To assess phylogenetic discordance and noise in our data, we used likelihood mapping (Strimmer and von Haeseler 1997) to estimate measures of tree-like, net-like and star-like evolution supported by each locus in the phylogenomic dataset for all 183 loci. Likelihood mapping was conducted in IQ-TREE by using the ‘lmap’ function with 5000 randomly drawn quartets, and scores for basins of attraction (where Regions 1, 2, 3 support tree-likeness; Regions 4, 5, 6 support net-likeness; Region 7 supports star-likeness) were evaluated for each locus alignment. To minimise the contribution of net-like and star-like topologies, locus alignments were then grouped into three subsets on the basis of percentage of fully resolved quartets (i.e. tree-likeness) at minimum values of 50, 60 and 70%. Maximum-likelihood phylogenies for each of these subsets were generated in IQ-TREE and branch support was estimated using UFBoot and SH-aLRT values with 1000 replicates. For this, alignments were automatically concatenated and analysed using the ‘−p’ option (to partition by locus). We also manually concatenated the alignments of each subset and viewed these as a NeighbourNet (Bryant and Moulton 2002) phylogenetic network in SplitsTree (ver. 4.17.0, see https://software-ab.cs.uni-tuebingen.de/download/splitstree/welcome.html; Huson and Bryant 2006) under default parameters (i.e. using uncorrected P distances and equal-angle splits).
To test support for the various possible resolutions of the polytomy, we conducted tree-topology tests. The maximum-likelihood (ML) tree produced from the phylogenomic alignment partitioned by locus was read into R, and all possible topological resolutions for the polytomy node were generated with the ‘resolveNodes’ function of the package phytools (ver. 1.0-3, see https://github.com/liamrevell/phytools; Revell 2012). We also generated an additional hard polytomy topology (i.e. truly multifurcating) by collapsing the short unsupported branches that formed the polytomy in the ML tree. To account for the potential influence of branch lengths on topology scores, all trees were treated as cladograms with branches set to a length of one. Topology tests were then run on this set of trees in IQ-TREE, by using the ‘−zw’ option to calculate log-likelihoods, estimating model parameters with ‘−n 1’, and conducting the bootstrap proportion test, unweighted and weighted Kishino–Hasegawa (Kishino and Hasegawa 1989) and Shimodaira–Hasegawa tests (Shimodaira and Hasegawa 1999), expected likelihood weights test (Strimmer and Rambaut 2002) and approximately unbiased test (Shimodaira 2002) by using 100 000 RELL (Kishino et al. 1990) replicates.
Results
Analyses of plastome data (phylogenomic alignment)
The phylogenomic alignment included 30 038 variable sites, of which 16 845 were parsimony informative (Table 3). Across all phylogenetic analyses of the phylogenomic alignment, no supported topological incongruence was recovered (Supplementary Fig. S1). The IQ-TREE analyses produced identical topologies and similar branch support across all partition schemes. The MrBayes analyses produced topologies with no supported incongruence. Across all analyses, and consistent with previous findings (Bayly et al. 2013; Appelhans et al. 2021), we found high support (posterior probability (PP): 1, UFBoot (UFB): 100, SH-aLRT (SH): 100) for the Eriostemon group, with Neoschmidia and Halfordia as the first successive divergences from the rest of the clade (see Fig. 3). Relationships in the Eriostemon group were mostly well resolved and fully supported, with the exception of a series of extremely short branches (coloured red on Fig. 3), effectively forming a polytomy in the backbone of the group in all analyses. The MrBayes and IQ-TREE phylogenies recovered four supported clades branching from this polytomy (see Fig. 3): Clade 1, including Drummondita, Geleznowia, Philotheca section Philotheca and Philotheca section Erionema; Clade 2, including Muiriantha and Philotheca section Cyanochlamys; Clade 3, including Correa, Leionema and Myrtopsis; Clade 4, including Asterolasia, Chorilaena, Crowea, Diplolaena, Eriostemon, Nematolepis, Phebalium and Philotheca section Corynonema. We note that topology of the MrBayes tree was slightly different from that of the IQ-TREE, with Clades 2–4 being resolved as a polytomy (hence the missing support value (‘−’) in Fig. 3) in an unsupported clade (PP: 0.53); but the low support for this clade still effectively rendered Clades 1–4 as a polytomy.
Item | Number of variable sites | Number of parsimony-informative sites | Number of constant sites | Total length (bp) | |
---|---|---|---|---|---|
Phylogenomic alignment | 30 038 | 16 845 | 76 847 | 106 885 | |
Supermatrix alignment | 30 057 | 16 895 | 76 657 | 106 714 |
Maximum-likelihood phylogeny of the Eriostemon group produced from IQ-TREE analysis of the unpartitioned ‘phylogenomic’ alignment of samples with full plastome data. UFboot and SH-aLRT support for branches are denoted by dots at nodes, where open circles denote 100% UFboot and 100% SH-aLRT support and closed circles denote 100% UFboot and <100% SH-aLRT support, and no dot present on the node indicates <100% for both metrics. Concordance factors are provided next to node dots, with gene concordance (gCF) positioned above site concordance (sCF). Posterior probabilities from the MrBayes 50% majority-rule consensus tree are superimposed to the left of the concordance factors in bold type at nodes where branch support is not maximal (i.e. <1; dashes occur where a branch was non-existent in the MrBayes tree). Short, unsupported branches that effectively form a polytomy in the backbone of the tree are coloured red. Red asterisks at nodes indicate branches that are well supported in our phylogeny but were unsupported in the plastid sequence phylogeny of Duretto et al. (2023). Sections of Philotheca are listed in grey, and are linked to corresponding taxa by dashed lines.
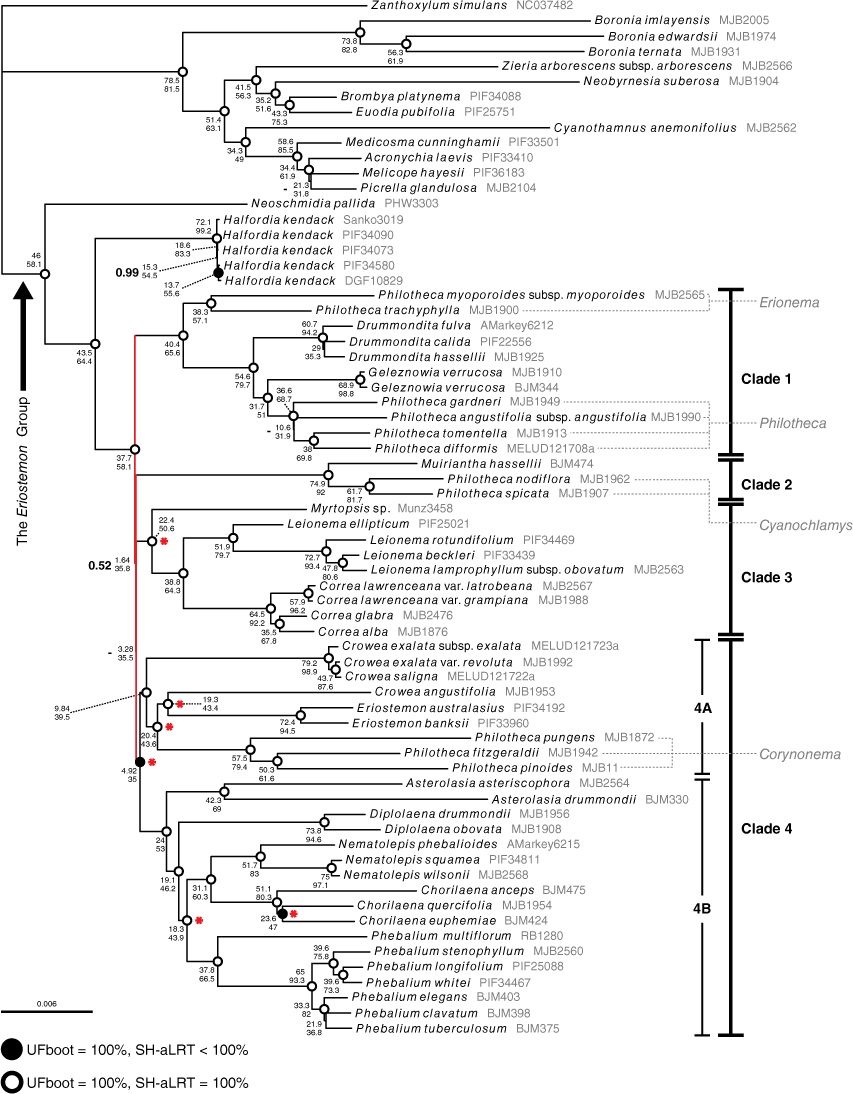
The phylogeny produced with ASTRAL recovered five supported clades branching from the polytomy, differing from the MrBayes and IQ-TREE phylogenies in lacking support for the unification of Eriostemon and its allies (Clade 4A in Fig. 3; Crowea, Philotheca section Corynonema) with Phebalium and its allies (Clade 4B in Fig. 3; Asterolasia, Diplolaena, Chorilaena, Nematolepis) (see Clade 4, Fig. S1). The ASTRAL polytomy test did not reject the null hypothesis that the backbone polytomy should not be treated as a true polytomy (Supplementary Fig. S2: P > 0.05 for all branches) and did not reject the collapsing of the branch uniting the Eriostemon alliance (i.e. Clade 4 A) and Phebalium alliance (i.e. Clade 4B) (P = 0.629). Concordance factors for the branch uniting the two Clade 4 alliances in the unpartitioned phylogenies are low, but all other support values are high (Fig. 3).
Within Clade 1, Philotheca section Erionema was resolved as sister to other members with high support (PP: 1, UFB: 100, SH: 100). Drummondita was placed sister to Geleznowia and Philotheca section Philotheca with high support, and Geleznowia and Philotheca section Philotheca were resolved as sister to each other with high support.
Clade 2 was resolved on a highly supported long branch, with Muiriantha sister to Philotheca section Cyanochlamys.
In Clade 3, Myrtopsis was placed sister to Correa and Leionema with high support. The monophyly of Correa and of Leionema was highly supported.
The largest clade, Clade 4, was resolved with high posterior probability and bootstrap support, but low gene and site concordance (PP: 1, UFB: 100, SH: 99.9, gCF: 4.92, sCF: 35). We found high support for a clade (Fig. 3, 4a) comprising Crowea, Eriostemon and Philotheca section Corynonema. Crowea was resolved as polyphyletic, with the two eastern Australian species, C. exalata and C. saligna, placed sister to the rest Clade 4A with high support. Crowea angustifolia, the sole western Australian species of the genus, was resolved sister to Eriostemon on a long branch with high support, and together this clade was placed sister to a monophyletic Philotheca section Corynonema with high support. The other taxa of Clade 4 (Asterolasia, Chorilaena, Diplolaena, Nematolepis, Phebalium) constitute a group that has been historically referred to as the Phebalium group (Wilson 1998b; Mole et al. 2004). In our analyses, these genera formed a well-supported clade (Fig. 3, 4b) that was placed sister to Clade 4A. Within the Phebalium group, Asterolasia was resolved as sister to the rest of the group with high support. Diplolaena was found to be sister to the remaining genera with high support. Also with high support, Nematolepis was resolved sister to Chorilaena. Phebalium was resolved sister to the clade of Chorilaena and Nematolepis with high support.
Phylogenetic relationships in Clade 1. Maximum-likelihood phylogeny produced from IQ-TREE analysis of the ‘supermatrix’ alignment of combined full plastome and Sanger sequences. UFboot support for branches is positioned above posterior probabilities from the MrBayes 50% majority-rule consensus tree of the same dataset. Relationships in other clades are identical to those in Fig. 3. Unsupported short branches in the backbone of the Eriostemon group have been manually collapsed to a polytomy. Asterisks denote samples represented only by Sanger sequence data. The MrBayes and IQ-TREE phylogenies differed in the placement of Philotheca angustifolia; this incongruence is shown by red-dashed branches that indicate the topology recovered by MrBayes (posterior probability value relevant to this is in red). Black triangle, Philotheca s.str. (largely equivalent to Philotheca sensu Wilson 1971); black square, Philotheca ‘Nigrostipulae’ (largely equivalent to Eriostemon section Nigrostipulae sensu Wilson 1970), as discussed in the text.
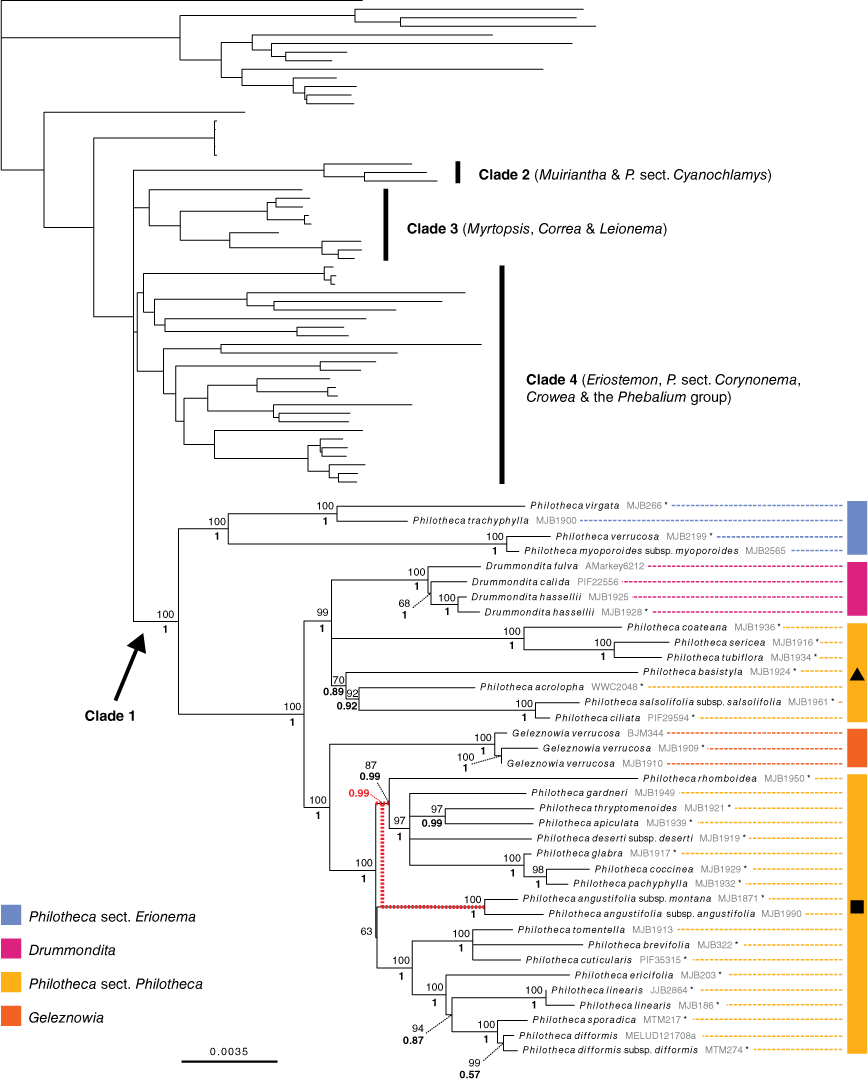
Analyses of combined plastome and Sanger data (supermatrix alignment)
The supermatrix alignment included 30 057 variable sites and 16 895 parsimony-informative sites (Table 3). The topologies recovered in both the IQ-TREE and MrBayes phylogenies were consistent with those produced by analyses of the phylogenomic alignment. No supported topological incongruence was present across the IQ-TREE and MrBayes phylogenies. The additional samples represented by Sanger sequence data in the supermatrix dataset were all placed in Clade 1 (i.e. with Philotheca section Erionema, Drummondita, Geleznowia, and Philotheca section Philotheca; see Fig. 4). As the supermatrix and phylogenomic alignment phylogenies differ only in Clade 1, the IQ-TREE phylogeny built from the supermatrix alignment (Fig. 4) is presented, with emphasis on relationships in Clade 1.
In Clade 1 Philotheca section Erionema was strongly supported as monophyletic and sister to all other taxa. Philotheca section Philotheca was recovered as polyphyletic, with P. coateana Paul G.Wilson, P. sericea (Paul G.Wilson) Paul G.Wilson, P. tubiflora A.S.George, P. basistyla Mollemans, P. acrolopha Paul G.Wilson, P. salsolifolia (Sm.) Druce and P. ciliata Hook. placed in a well-supported clade with Drummondita. The other members of Philotheca section Philotheca formed a highly supported clade sister to Geleznowia. The single, but poorly supported, topological difference between the IQ-TREE and MrBayes phylogenies was the position of Philotheca angustifolia within section Philotheca (PP: 0.99 v. UFB: 63; indicated in Fig. 4).
Investigation of the Eriostemon group polytomy
Our approach identified the optimal number of gene tree clusters in our data as three. Cluster 1 contained 72 loci (33 protein-coding, 39 non-coding) with a mean length of 955 bp, and a mean number of parsimony-informative sites of 141. Cluster 2 contained 50 loci (21 protein-coding, 29 non-coding) with a mean length of 276 bp, and a mean number of parsimony-informative sites of 44. Cluster 3 contained 26 loci (17 protein-coding, 9 non-coding) with a mean length of 378 bp, and a mean number of parsimony-informative sites of 66. Re-estimating the phylogeny for each of the three clusters produced trees with no supported incongruence between, for both IQ-TREE (Supplementary Fig. S3) and ASTRAL phylogenies (see files under Data availability). RAxML gene trees from Clusters 1 and 2 were significantly longer than those in Cluster 3 (Dunn’s test; Bonferroni adjusted P = 8.99 × 10−6 and P = 5.52 × 10−4 respectively; Supplementary Fig. S4). Bootstrap support was significantly different among all clusters, with support values in Cluster 1 being markedly higher than those in Clusters 2 and 3 (Dunn’s test; Bonferroni adjusted P = 2.97 × 10−13 for C1–C2, P = 2.31 × 10−21 for C1–C3, P = 1.87 × 10−3 for C2–C3; Fig. S4).
Across all loci, the mean percentage of fully resolved quartets (i.e. quartets falling into Regions 1, 2 and 3 of the likelihood-mapping plot) was 53%. The means for partly resolved quartets (Regions 4, 5 and 6) and unresolved quartets (Region 7) were 4 and 43% respectively. The subset of loci with >50% fully resolved quartets contained 102 loci with a mean length of 851 bp, and a mean number of parsimony-informative sites of 140. The subset of loci with >60% fully resolved quartets contained 63 loci with a mean length of 1056 bp, and a mean number of parsimony-informative sites of 178. The subset of loci with >70% fully resolved quartets contained 22 loci with a mean length of 1490 bp, and a mean number of parsimony-informative sites of 302.
Phylogenetic analysis of each of the subsets resulted in trees with identical topologies that were congruent with the phylogeny produced in the analysis of the unpartitioned full dataset (i.e. Fig. 3). In general, all subset trees had slightly lower branch support than the full-dataset tree. Among subset trees, the >70% tree had the lowest branch support, with exception to the placement of Philotheca angustifolia sister to P. tomentella and P. difformis with low-to-moderate support (UFBoot: 91; SH-aLRT: 82.9). The >50 and >60% trees did not resolve the position of this species. Despite the removal of potentially noisy or discordant loci, none of the subset trees contained a supported resolution for the backbone polytomy; the >60% subset tree displayed the highest support for short branches of the polytomy, but these branches were still unsupported and resulted in star-like resolution of the polytomy in our network analysis (Fig. 5b). We found a significant difference in the number of resolved quartets between the three gene tree clusters from the tree space analysis (one-way ANOVA test; F(2,145) = 18.82, P = 5.39 × 10−8; Kruskal–Wallis rank sum test; H = 40.437, d.f. = 2, P = 1.657 × 10−9). The mean number of resolved quartets for Clusters 1, 2 and 3 was 3019 (σ = 586), 2434 (σ = 541) and 2213 (σ = 1030) respectively, indicating that Cluster 1 contained the most phylogenetically informative loci on average.
Results of the likelihood-mapping analysis of loci. (a) Plot of phylogenetic informativeness of individual loci, where a higher percentage of fully resolved quartets for a locus indicates greater support for tree-like evolution. Dashed lines on the plot indicate the three cut-off values that were tested for potentially improving phylogenetic resolution of the polytomy (i.e. 50, 60 and 70%). Bars along the x-axis denote the cluster that each locus was placed in during the analysis of tree space; some loci were excluded from the tree-space analysis, owing to incomplete representation of samples. (b) Phylogenetic network (NeighborNet) showing splits of the backbone polytomy; constructed from a concatenated alignment of all loci with >60% of quartets fully resolved. For Crowea, EA, eastern Australia; WA, western Australia.
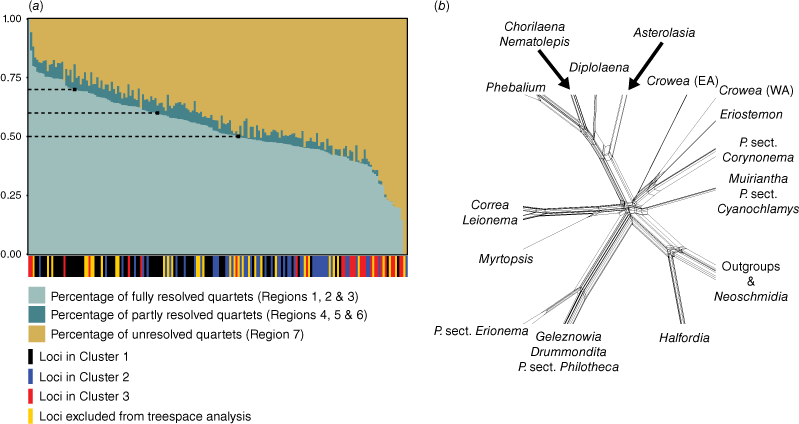
Owing to high UFBoot and posterior probability support for four clades forming the backbone polytomy, our tree-topology testing investigated support for the 15 possible relationships among these clades (Fig. 6a). Log-likelihood values for each topology ranged from −494 970.83 to −494 975.48. Expectedly, the topology of the most likely tree used as input was found to have the highest log-likelihood (−494 970.83); this topology was identical to the 15th possible resolution of the polytomy (Fig. 6a). Five topologies were found to have slightly higher log-likelihoods than the others (Fig. 6b: Topologies 15, 5, 10, 13, 14). The three most likely topologies (15, 5, 10) were the only topologies to resolve the Clade 1 sister to the rest of the group. Clades 1 and 2 were grouped sister to Clades 3 and 4 in Topology 13, and in Topology 14 Clade 2 was sister to the rest of the group, with Clade 1 being sister to Clades 3 and 4. The AU test rejected four topologies (P < 0.05): 7, 9, 12 and the hard polytomy. The hard polytomy was found to be one of the least likely topologies, having a low log-likelihood score (−494 975.27) and being statistically supported by only the unweighted and weighted SH tests (P = 0.225 and P = 0.648), which did not reject any topologies (P-values greater than 0.05 for all topologies). The bootstrap proportion and equal likely weights test did not place the hard polytomy topology in the 95% confidence set of trees. Summary statistics from topology testing are provided as Supplementary Table S4.
Results of topology tests for the backbone polytomy in the Eriostemon group. (a) The 15 possible resolutions of the polytomy (for the four supported clades) that testing was conducted on, ordered by most likely to least likely from left to right, top to bottom (note: the hard polytomy resolution is not depicted). (b) Plot of log likelihoods for each possible topology. The topology matching the most likely tree is denoted by a star, and the hard polytomy topology is denoted by a triangle.
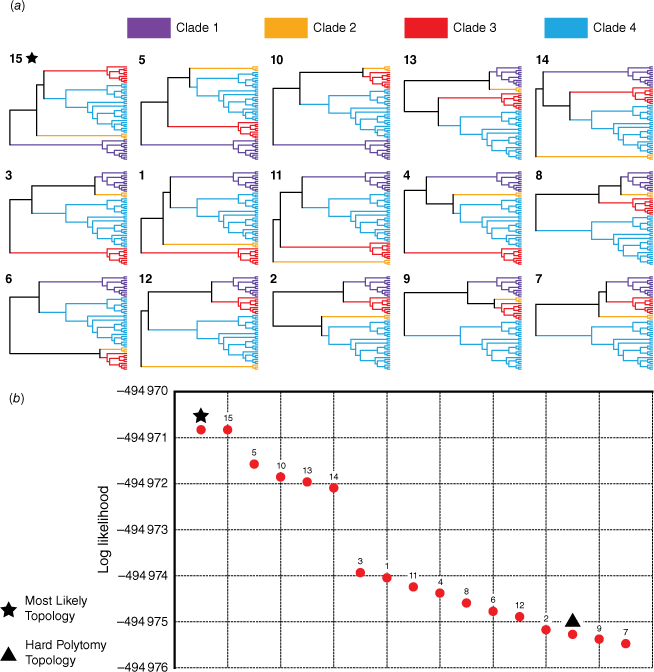
Discussion
Generic relationships in the Eriostemon group
The current study was conducted concurrently with a similar study by Duretto et al. (2023), who also investigated relationships in the Eriostemon group. Duretto et al. (2023) focussed primarily on relationships in the Phebalium group (Clade 4B in our analyses) and Leionema, sampling comprehensively across those genera by using five markers (5145 bp in total; plastid markers psbA–trnH, trnL–trnF, rbcL; nrDNA markers ITS, ETS). With their smaller genetic dataset but more thorough taxon sampling of the group, they confirmed the monophyly of Asterolasia, Diplolaena, Leionema, Nematolepis and Phebalium (Duretto et al. 2023). This is congruent with our results, although our sampling of these genera is not appropriate for commenting on their monophyly. The results of our analyses are mostly congruent with those of Duretto et al. (2023) and support the taxonomic changes made by those authors, namely, the transfer of Rhadinothamnus (=C. anceps and C. euphemiae in our analyses) to Chorilaena, and Microcybe (=P. multiflorum in our analyses) to Phebalium. Our larger genetic dataset has further clarified relationships among some genera by providing maximal support for branches previously unresolved (<0.95 PP in the ptDNA phylogeny of Duretto et al. 2023; indicated on Fig. 3). The following sections discuss some relationships, supported here, that are noteworthy; relationships in the Phebalium group are not discussed here because they have already been thoroughly discussed by Duretto et al. (2023).
We provide high support for a clade of Correa, Leionema and Myrtopsis, three genera that previous studies have been unable to confidently place (Bayly et al. 2013; Appelhans et al. 2021); this group was recovered as an unsupported clade by Duretto et al. (2023). This clade contains probably the most varied morphological characters in the Eriostemon group. The recovery of a sister relationship between Leionema and Correa is congruent with the plastid sequence phylogeny of Duretto et al. (2023) and is of note because the two genera differ vastly morphologically. Correa is unique in the Eriostemon group in possessing consistently 4-merous flowers that are mostly tubular and bird-pollinated (except for C. alba Andrews, which is nested among bird-pollinated species) (Armstrong 1979; French et al. 2016). Although bird pollination occurs in other taxa (e.g. some Leionema, Nematolepis and Philotheca), the flowers of all other genera in the group are 5-merous (with the exception of Philotheca virgata (Hook.f.) Paul G.Wilson). Correa is also distinct in possessing leaves that are oppositely arranged, although this feature also occurs in Myrtopsis. All other members of the Eriostemon group have alternate leaves. Consequently, the order of relationships among Correa, Leionema and Myrtopsis that we have recovered, (Myrtopsis (Correa, Leionema)), implies either a single transition to opposite leaves at the stem of the clade and a subsequent reversal to alternate leaves in Leionema, or separate independent transitions to opposite leaves in Myrtopsis and Correa. The placement of the New Caledonian endemic Myrtopsis firmly within the Eriostemon group is consistent with several previous studies (Bayly et al. 2013; Appelhans et al. 2021; Baker et al. 2022; Duretto et al. 2023).
Historically, the relationship of Myrtopsis with other genera of Austral-Pacific Rutaceae has been difficult to deduce from morphology. On the basis of morphological cladistic analyses, Armstrong (1991) resolved the genus as sister to the Eriostemon group (minus Correa, which was placed in the Boronia clade), although this placement was equivocal owing to limited availability of plant material. This contrasted with Hartley (1995), who considered Myrtopsis most closely related to Boronella Baill. (=Boronia Sm.), Euodia, Brombya and Medicosma, and separated the genus from the rest of tribe Boronieae on the basis of embryo shape and cotyledon width. Certain elements of the distinct morphology of Myrtopsis could be related to adaptation to New Caledonian habitats with nutrient-poor, ultramafic soils and comparatively higher temperatures and humidity than in the southern half of Australia, where the majority of species in the Eriostemon group occur.
The presence of Myrtopsis only in New Caledonia is interesting biogeographically. Evidence exists for possible land connections between Australia and New Caledonia into the Paleocene and Eocene ~60–35 million years ago (Ladiges and Cantrill 2007), meaning that a vicariance explanation cannot be ruled out should the divergence of Myrtopsis pre-date this. Time-calibrated phylogenies have tentatively estimated the divergence of Myrtopsis from the Oligocene to the mid-Miocene ~30–15 million years ago (Bayly et al. 2013; Joyce et al. 2023), implicating long-distance dispersal to New Caledonia from Australia after re-emergence of the main island during the Paleocene–Eocene (37 ± 3 million years ago; Grandcolas 2017). This is in line with the crown ages of most New Caledonian clades across the tree of life (Nattier et al. 2017). However, Bayly et al. (2013) stated that their divergence-date estimates should be treated with caution because of a limited availability of fossils within Rutaceae for calibration; they highlighted that trial analyses conducted with fewer fossil calibrations had the effect of producing much younger age estimates. Hence, the divergence of Myrtopsis in that estimation may appear younger than it should, and a vicariance explanation for the split should not yet be discounted on the basis of the current understanding of phylogenetic relationships and lack of consensus from divergence-dating analyses.
The placement of Crowea, Eriostemon and Philotheca section Corynonema in a highly supported clade (Clade 4A), together with strong support for relationships in that clade, improved on previous understanding of the ptDNA relationships of these taxa. Duretto et al. (2023) also resolved these three taxa in a well-supported clade in two of their analyses, but found somewhat conflicting relationships between the taxa according to different datasets; they found weak support for a sister relationship between Eriostemon and Crowea in their combined nrDNA–ptDNA phylogeny, weak support for a sister relationship between Philotheca section Corynonema and Crowea in their ptDNA phylogeny, and the taxa were not resolved in a clade in their nrDNA phylogeny. We have not identified obvious morphological synapomorphies that unite all three groups, but several morphological studies have proposed a sister relationship between Crowea and Eriostemon that is incongruent with our placement of Eriostemon (and Crowea angustifolia Sm.; see next paragraph for discussion of that species) as sister to Philotheca section Corynonema (Armstrong 1991; Bayly 2001). Crowea and Eriostemon differ from the rest of the Eriostemon group in having petals with at least three main veins (rather than one main vein) originating from their base (Bayly 2001). Armstrong (1991) also considered Crowea and Eriostemon united by staminal filaments that are arranged pyramidally over the ovary at anthesis, although this was questioned by Bayly (2001), who argued that the feature is not as striking in Eriostemon, and that staminal arrangement in Eriostemon is not dissimilar to some species of Philotheca (=Eriostemon section Nigrostipulae Paul G.Wilson). In addition, chromosome numbers for Crowea (n = 19) and Eriostemon (n = 17) are unique in the Eriostemon group, where n is most commonly 14 (Asterolasia, Geleznowia, Drummondita, Muiriantha, Philotheca sectin Philotheca, P. section Erionema, P. section Cyanochlamys, some Diplolaena) or 16 (Correa, Leionema, Nematolepis, Phebalium) (Smith-White 1954; Armstrong 1991; Stace and Armstrong 1992; Bayly 2001). Chromosome numbers for species in Philotheca section Corynonema are unknown, and future cytological investigation of this group would provide valuable insight into how well the cytology of this section aligns with Crowea and Eriostemon.
Our recovery of Crowea as polyphyletic in ptDNA analyses is perhaps not surprising. Duretto et al. (2023) found support for the monophyly of Crowea to vary between nrDNA and plastid datasets, with ptDNA suggesting that the genus may be non-monophyletic because of the only western Australian species, C. angustifolia (using different accessions to the current study), forming a polytomy with the eastern Australian Crowea and Eriostemon. Our results have improved the clarity of ptDNA relationships in this group and confirmed that ptDNA suggests Crowea is non-monophyletic with the placement of C. angustifolia as sister to Eriostemon with high support. However, our phylogenetic analyses of nrDNA sequences (see the Supplementary ‘Methods and results’ section) mirror the findings of Duretto et al. (2023) and suggest the genus is monophyletic. Such cytonuclear discrepancy may arise from one, or a combination of, incomplete lineage sorting (ILS), horizontal gene transfer and organellar genome capture (Rieseberg and Soltis 1991; Tsitrone et al. 2003; Toews and Brelsford 2012). Given that both Eriostemon species occur only in eastern Australia, it is unlikely that their sister placement to C. angustifolia in ptDNA trees is the result of the last two processes, as they require gene flow between the genera (or their common ancestors) to occur; on the basis of the current distribution of taxa, it is simpler to infer that there has been no historical reconnection between C. angustifolia and Eriostemon, rather than infer that there has been exclusive connectivity between the two (across the whole of Australia) in the absence of gene flow with the other species of Crowea. Although we cannot rule out the latter scenario, we feel a more likely explanation involves ILS of the plastome of C. angustifolia, because this matches the simpler scenario outlined above and is consistent with ILS having a greater influence on plastid markers due to larger effective population sizes, and thus longer coalescence times than for nrDNA markers that are subject to concerted evolution (Buckler and Holtsford 1996; Clowes et al. 2022). Further work is required to deduce the cause of this discordance.
The placement of Philotheca section Cyanochlamys sister to Muiriantha in Clade 2 is consistent with the findings of Duretto et al. (2023). These taxa are quite distinct from each other morphologically, and were speculatively thought to be allied to Philotheca (for P. section Cyanochlamys) and the Phebalium group (for Muiriantha) on morphological grounds (Wilson 1970). The only species of Muiriantha, M. hassellii (F.Muell.) C.A.Gardner, and both species of P. section Cyanochlamys, P. spicata (A.Rich.) Paul G.Wilson and P. nodiflora (Lindl.) Paul G.Wilson, are endemic to south-western Australia, so this relationship is geographically sensible. Muiriantha hassellii has pendulous flowers with imbricate yellowish-green petals that form an elongated tube (Fig. 7a), whereas the flowers of Philotheca section Cyanochlamys are borne erectly, with petals spreading and ranging from pink to blue in colour (Fig. 7b, c).
Flowers, carpels (with pitted surfaces), and fasciculate stem hairs of Muiriantha and Philotheca section Cyanochlamys (voucher numbers indicated in brackets): (a, d, f) Muiriantha hassellii [MJB 2574, MELUD155083a], (b, e, h) Philotheca nodiflora subsp. lasiocalyx [b, e, MJB 108, MELU; h, MJB 1962, MELUD105840a], (c, f, i) Philotheca spicata [c, f, MJB 9, MELU; i, MJB 10, MELU]. Scale bars: 500 μm, in micrographs of carpels (inset close-up images of pits are not to scale), and 100 μm, in micrographs of hairs (arrows indicate fasciculate hairs in h, i that are obscured by other hairs).
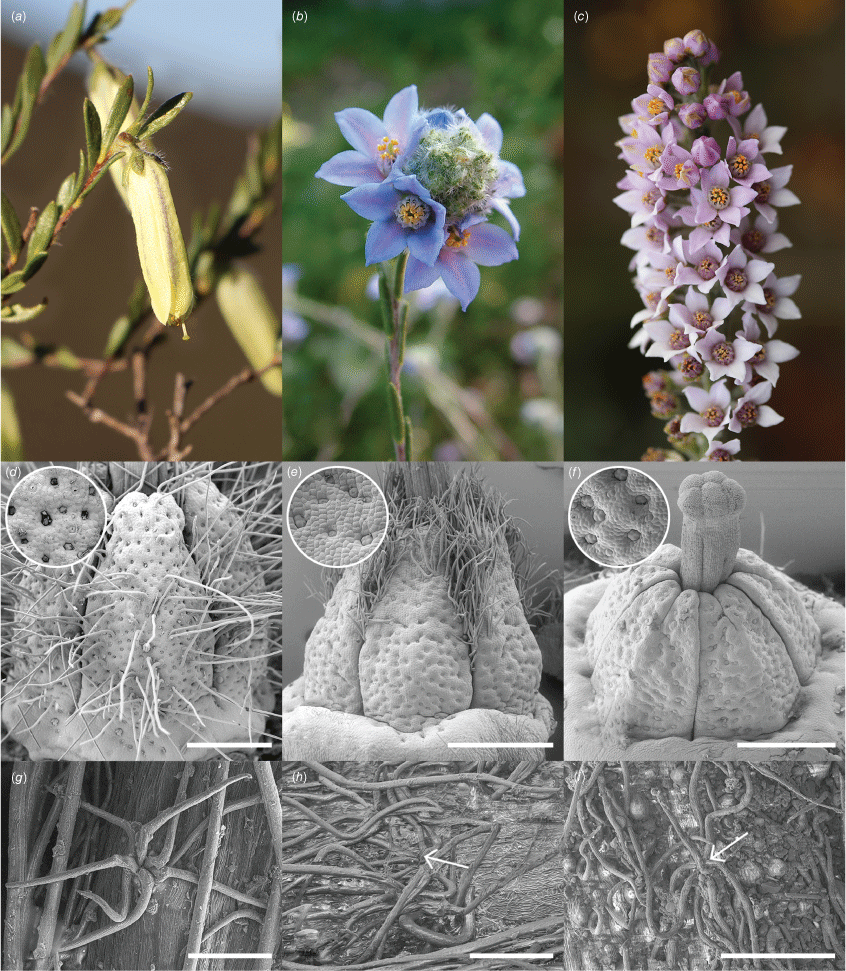
On the basis of Duretto et al. (2023) and our nrDNA analyses (see the Supplementary ‘Methods and results’ section), it is apparent that the close relationship of Philotheca section Cyanochlamys and Muiriantha is consistent across nrDNA and ptDNA. However, in contrast to our ptDNA results, our analyses of nrDNA resolved Muiriantha nested inside P. section Cyanochlamys, with very high support (see the Supplementary ‘Methods and results’ section). Duretto et al. (2023) found the same discrepancy in the position of Muiriantha between ptDNA and nrDNA datasets, but the nesting of Muiriantha in P. section Cyanochlamys in their nrDNA phylogeny was not well supported.
Despite obvious morphological differences between Philotheca section Cyanochlamys and Muiriantha, they are broadly similar in having a subshrub habit and leaves with a somewhat papery texture. Further morphological examination by the authors has also shown similarities in carpel surfaces and branchlet indumenta (Fig. 7).
In Muiriantha and P. section Cyanochlamys, the abaxial surface of the carpels is conspicuously pitted with small glands (Bayly 2001; Fig. 7d–f). This feature is otherwise known only in Philotheca pinoides (section Corynonema) (Bayly 2001) and is, therefore, considered apomorphic for Muiriantha and section Cyanochlamys in the context of major clades of the Eriostemon group.
In Philotheca section Cyanochlamys, two types of branchlet hairs are found (Bayly 2001). The first type is long (~0.6 mm), silky, distally ascending and somewhat appressed, and is fasciculate (having multiple hairs arising from a single base) but not spreading; these hairs are always present in P. nodiflora, and are sometimes absent in P. spicata. The second type is fasciculate–stellate, with multiple hairs arising from a single base that spread out in a stellate manner, and occurs in both species of P. section Cyanochlamys (Bayly 2001). Muiriantha also possesses two types of hairs; the first is similar to the long, silky, appressed hairs of P. section Cyanochlamys, but differs in being singular (i.e. one hair, not multiple from a single base), the second is fasciculate hairs that are the same as those of P. section Cyanochlamys. Fasciculate hairs of this type (Fig. 7g–i) are an apparent synapomorphy for Clade 2, as they do not occur in any other members of the Eriostemon group.
Finally, from comparing descriptions of Philotheca sections Cyanochlamys and Muiriantha (Wilson 1970, 2013a, 2013b), it is apparent that seed features between these taxa are superficially similar. Preliminary investigations of seeds in these taxa by the authors has confirmed that they share a subreniform shape, linear hilum and sub-basal raphe, but it remains unclear whether these characteristics are synapomorphic or plesiomorphic. More detailed investigations of seed morphology may clarify whether or not these similar features should be treated as synapomorphic, and provide further characters that could be used to assign these taxa to a morphologically diagnosable taxonomic group (e.g. subtribe).
Our well-supported recovery of Clade 1, containing Philotheca section Erionema, P. section Philotheca, Drummondita and Geleznowia, is consistent with results of Duretto et al. (2023), and was also recovered with low support by Appelhans et al. (2021). Within this clade, the monophyly and divergence of Philotheca section Erionema, placed as sister to the other taxa, is supported by several morphological synapomorphies (Bayly 2001; Batty et al. 2022), including anther and seed characters. In particular, the seeds of P. section Erionema are unique in the Eriostemon group and readily recognisable in being ellipsoid and laterally flattened with a linear hilum on the adaxial face and having a basal raphe and chalazal region (Fig. 8; Wilson 1998a; Bayly 2001). In contrast, seeds of Philotheca section Philotheca, Drummondita and Geleznowia (Fig. 8), which share a strong morphological resemblance in comparison to other members of the Eriostemon group, are more or less reniform, substantially thicker than their length, have a smaller hilum that is round to deltoid and located, together with the raphe, on the adaxial face of the seed.
Seed morphology in Clade 1, showing the adaxially central raphe in Philotheca section Philotheca, Drummondita and Geleznowia, and the basal raphe in Philotheca section Erionema. (a–c) Seeds typical of P. section Philotheca [P. linearis; G.J. White s.n., NE 52727]. (d–f) Seeds typical of Drummondita [D. longifolia; H. Demarz 10361, PERTH 959707]. (g–i) Seeds typical of Geleznowia [G. verrucosa; L. Broadhurst 14, PERTH 5547822]. (j–l) Seeds typical of P. section Erionema [P. verrucosa; MJB 249, HO523410]. (a, d, g, j) Lateral views. (b, e, h, k) Adaxial views. (c, f, i, l) Longitudinal sections through the raphe. (a, g) Drawn with the placental portion of endocarp still attached to the seed; for all other drawings the placental endocarp was removed. Drawings are modified from Bayly (2001) and are not to scale.
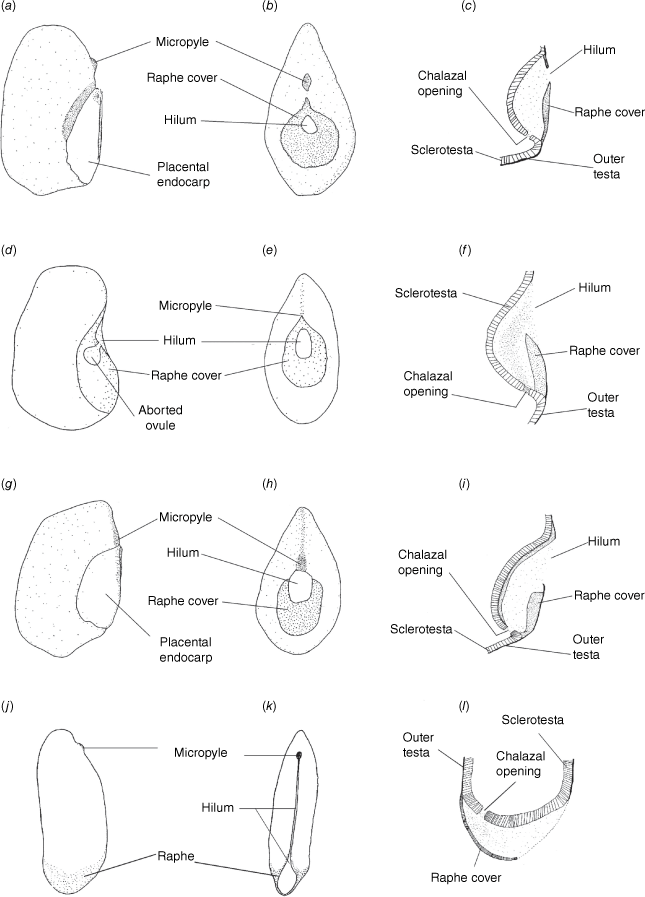
Our supermatrix approach expands on the results of previous molecular studies with more comprehensive sampling from Philotheca section Philotheca. Our results show Philotheca section Philotheca as polyphyletic, with members of this section falling into two separate clades, one including Drummondita and one sister to Geleznowia (Fig. 4).
The species of section Philotheca that group with Drummondita constitute (with the exception of P. coateana) an assemblage that, prior to the revision of Wilson (1998a), was considered the entirety of Philotheca. This narrow concept of Philotheca (referred to hereafter as Philotheca s.str.; Fig. 4, black triangle), first circumscribed by Bentham (1863), included only species with 10 fertile, monadelphous stamens and a spreading corolla. Drummondita also has monadelphous stamens, and this feature presumably led Mueller (1869) to include that genus in Philotheca. However, Drummondita was reinstated at generic rank by Wilson (1971) on the basis of key differences including stamen fertility (only five fertile in Drummondita), anther attachment (dorsifixed in Drummondita, versatile in Philotheca), petal texture (glumaceous in Drummondita, soft in Philotheca) and carpel apex (unbeaked in Drummondita and beaked in Philotheca); as a result, Philotheca (sensu Wilson 1971) returned to its original, narrow circumscription. Wilson (1998a) expanded Philotheca to include Philotheca s.str., plus all species previously included by Wilson (1970) in Eriostemon section Nigrostipulae.
Among members of Philotheca s.str., fusion of the staminal filaments is present in Philotheca salsolifolia, P. ciliata, P. basistyla, P. tubiflora, P. acrolopha and P. sericea (Bayly 2001), but the degree of fusion varies among these species. In particular, the filaments of P. acrolopha and P. sericea are fused only very minutely at the base (Bayly 2001). In our analyses, the only species to be placed in this clade that does not have fused filaments is Philotheca coateana, which was not considered part of Philotheca s.str. by Bayly (2001); the free nature of filaments in this species was verified in the current study by using scanning electron microscopy (see Supplementary Fig. S5).
The remaining members of Philotheca section Philotheca that are not part of Philotheca s.str. are equivalent to Eriostemon section Nigrostipulae sensu Wilson (1970) (referred to herein as Philotheca ‘Nigrostipulae’; Fig. 4, black square). In his revision of Eriostemon, Wilson (1998a) recognised the similarity of Geleznowia to his newly circumscribed Philotheca section Philotheca and contemplated that the arrangement he presented may render Philotheca paraphyletic with regards to Geleznowia. In particular, Wilson (1998a, 2013a) noted that Geleznowia and some members of Philotheca section Philotheca (including Philotheca s.str. and Philotheca ‘Nigrostipulae’) possess filiform sclereids, similar seeds and the same chromosome number. Although Geleznowia shares these morphological features with Philotheca section Philotheca in the broad sense (sensu Wilson 1998a), our recovery of a sister relationship specifically between Geleznowia and Philotheca ‘Nigrostipulae’ is not supported by any morphological synapomorphies that we can identify, probably because of the highly derived morphology of Geleznowia and a high degree of morphological homoplasy in Clade 1.
Interpreting the backbone polytomy in the Eriostemon group
The amount of sequence data employed in our analyses represents a ~14-fold increase in dataset size compared with the most recent other studies on the group (106 885 bp in our phylogenomic alignment v. 7579 bp in Appelhans et al. 2021 and 5145 bp in Duretto et al. 2023). Even with this large dataset, we were unable to resolve backbone relationships between four supported major lineages of genera (Fig. 3). Across all analyses, these lineages were arranged on very short, unsupported branches that we have treated as a polytomy. Short divergences in molecular phylogenies may be explained by both biological and experimental causes. Biologically, polytomies can be the result of true multifurcations (so-called hard polytomies) caused by rapid radiation and simultaneous divergence events. However, polytomies may arise as a result of reaching the limit of resolution in the estimated tree (so-called soft polytomies) caused by using an insufficient amount of sequence data or sequence variation, conflicting data, or inappropriate methods of analysis (Slowinski 2001; Whitfield and Lockhart 2007; Lin et al. 2011; Sayyari and Mirarab 2018).
In ptDNA, conflicting phylogenetic signal may be attributed to topological incongruence among gene trees, noise introduced by site saturation, or applying substitution models uniformly across genes with differing rates of evolution.
The first of these causes belongs to the species tree paradigm (Doyle 2021), in which systematists infer species trees from gene trees under the multispecies coalescent (MSC) model that assumes free recombination between unlinked genes (Edwards 2009). Because of this assumption, coalescence-based approaches have generally been applied to studies utilising unlinked nuclear genes. However, several studies have reported conflicting phylogenetic signals among plastid genes (e.g. Zeng et al. 2014; Foster et al. 2018; Gonçalves et al. 2019), and the topic of whether these methods are suitable for analysing plastid loci (which have historically been treated as linked; Doyle 1997) has been subject to recent debate (Gonçalves et al. 2020; Doyle 2021). Although our analysis of tree space identified three different gene-tree clusters, estimation of the cluster phylogenies produced congruent topologies that differed mainly in their levels of branch support; thus, we found no evidence for conflicting signals among gene trees. This may reflect a failure of Robinson-Fould distances to adequately identify meaningful phylogenetic similarity (see Smith 2022), or simply show that there is no strong conflict among gene trees in each cluster. Instead of differences in tree topology among clusters, the identification of three gene-tree clusters may be the result of differing levels of phylogenetic informativeness across gene trees, with Cluster 1 containing, on average, the most informative genes with generally better-resolved topologies than for Clusters 2 and 3. We attribute the generally lower branch-support values displayed by our ASTRAL phylogeny to the lower resolving power of individual genes v. concatenated genes (Doyle 2021), rather than conflicting gene-tree topologies (this is also supported by gene discordance factors for the tree in Fig. 3; mean gCF: 41.9, mean gDF1: 5.8, mean gDF2: 5.6 across all branches; see Data availability for file).
Second, we are able to dismiss noise caused by site saturation as a potential source of conflict on the basis of the persistence of the polytomy in the analyses of the likelihood mapping subsets (with noisy loci removed), the DNA alignment partitioned by codon position, and the translated CDS alignment (Supplementary Fig. S6). Similarly, if rate variation among loci were a contributor of conflict, then our IQ-TREE analysis partitioned by locus would have produced a result different from that from the unpartitioned analysis. Hence, we consider it unlikely that conflicting phylogenetic signal is a significant cause of the short, unsupported branches of the polytomy.
Previous molecular phylogenies of Rutaceae have resolved deeper relationships than that of our polytomy with far less ptDNA sequence data and comparable sequence variation (e.g. Groppo et al. 2008, 2012; Bayly et al. 2013; Appelhans et al. 2021). Several studies have also shown that lower-level relationships can be resolved using less data (e.g. Barrett et al. 2014; Bayly et al. 2016; Duretto et al. 2020, 2023). On the basis of these precedents, our dataset should be appropriate for resolving relationships at the taxonomic level of the polytomy. However, some biological characteristics of the plastome may render it inappropriate for resolving divergences that occurred over a short period of time. In plants, the plastome evolves at a relatively slow rate, at least half that of the nuclear genome (Wolfe et al. 1987). Genomes that evolve at slower rates are less capable of accumulating phylogenetically informative evolutionary changes during rapid radiations. In addition, lineage sorting is expected to progress more rapidly in ptDNA than in nDNA because of the smaller effective population size of the plastome (generally ¼ that of nuclear genes, with the exception of regions such as nuclear ribosomal DNA that are subjected to concerted evolution; Buckler and Holtsford 1996; Palumbi et al. 2001). Because of these features, the plastome may not be as useful as the nuclear genome for investigating lineages that have rapidly radiated, because there is less time for phylogenetically informative mutations to accumulate before lineages are sorted. If rapid radiation has occurred in the distant past, as is potentially the case in the Eriostemon group, this problem is likely to be compounded by subsequent lineage-specific mutations that can mask phylogenetically informative changes accumulated during the radiation (Whitfield and Lockhart 2007).
Our ASTRAL polytomy test was unable to reject that the polytomy is not a true multifurcation (P > 0.5 for all polytomy branches). However, as this test relies on calculating gene-tree topology likelihoods under the MSC model, the application of the test to our dataset of plastome loci is probably inappropriate (owing to divergence from the MSC model), and hence this result should be interpreted cautiously (Sayyari and Mirarab 2018; Doyle 2021).
Results from our tree-topology tests are likely to be more reliable. These showed a trend of higher support for topologies with Clade 1 as sister to the rest of the Eriostemon group, and low support for a multifurcating topology, suggesting that relationships between these lineages are perhaps more likely to be bifurcating rather than multifurcating.
The true nature of relationships around the backbone polytomy in the Eriostemon group remains difficult to answer with the current dataset, and we suggest that further investigation using more appropriate data, in particular, multi-locus nuclear DNA markers, is required before any conclusions are made regarding the hard or soft status of the polytomy. Clarification of the polytomy, and its hard or soft status, will also allow for interpretation of the higher-level branching order and evolution of the group.
Together, the clades that make up the backbone polytomy in the Eriostemon group (i.e. Clades 1–4) contain 16 genera (13 Australian) and ~204 species (~194 Australian) that include a large proportion of Australia’s Rutaceae diversity (total ~42 genera, ~490 spp.). Taxa in the Eriostemon group account for nearly all of the Rutaceae that occur on low-nutrient soils in dry sclerophyll communities in the south-east and south-west of Australia (Hartley 1995); the only genera outside this group that occur in similar habitat are Boronia (~125 spp. in Australia), Zieria (~63 spp. in eastern Australia), Neobyrnesia (1 sp. in N Northern Territory) and Cyanothamnus (23 spp. in Australia). Because of this, they are an important component of the Australian flora.
The phylogenetic depth at which the polytomy occurs means that it is largely irrelevant to the taxonomic delimitation of genera but may be pertinent to higher-level classification. Currently, revisions of tribal and subtribal classification are needed for Rutaceae (Appelhans et al. 2021). A new tribal classification would probably apply above the level of the polytomy and, hence, not require its resolution, but a robust future subtribal classification would certainly benefit from the resolution of the branching order of Clades 1–4. Subtribes in the Eriostemon group have been largely neglected since Engler (1931), who placed the contemporary genera (Muiriantha was not yet described) in the Eriostemon group across the following four subtribes: Eriostemoninae (including Asterolasia, Crowea, Drummondita (as a section of Philotheca), Eriostemon, Geleznowia, Leionema (as a section of Phebalium), Phebalium and Philotheca), Nematolepidinae (Chorilaena and Nematolepis), Correinae (monotypic, including Correa) and Diplolaeninae (monotypic, including Diplolaena). Under this scheme, Eriostemoninae is polyphyletic. On the basis of our phylogeny, one could propose a new system that recognises each of the major lineages of the group (i.e. Clades 1–4) as separate subtribes. Alternatively, subtribe Eriostemoninae could be split into several different subtribes so as to retain Correinae, Diplolaeninae and Nematolepidinae. In any case, a newly proposed classification should be constructed with consideration of relationships across the whole of the Rutaceae to ensure that taxonomic ranks are applied in a consistent manner, and should also consider the morphological diagnosability of subtribes, something that the current study does not thoroughly do.
Beyond taxonomic classification, resolving the branching order of the polytomy would prove useful to studies focussed on macroevolution and biogeography. In terms of morphology, the Eriostemon group displays multiple characters of biological and ecological interest that have evidently experienced state transitions (e.g. in floral features and phyllotaxy); studies investigating the evolutionary histories of characters by using methods that require bifurcating phylogenetic frameworks, such as ancestral-state reconstructions, would be enabled by resolution of the polytomy. Biogeographically, the Eriostemon group is noteworthy for having multiple genera that are disjunct between south-western and south-eastern Australia (e.g. Phebalium, 16 spp. in south-west, 22 spp. in south-east; Nematolepis, 1 sp. in south-west, 6 spp. in south-east; Philotheca section Erionema, 1 sp. in south-west, 14 spp. in south-east; Asterolasia, 5 spp. in south-west, 14 spp. in south-east). This distribution pattern occurs in many plant taxa and has been attributed to vicariance associated with marine inundations of south-central Australia and subsequent edaphic and climatic barriers during the mid-Miocene (~16–14 million years ago; Crisp and Cook 2007; Ladiges et al. 2010, 2012). Compared with other species-rich families in Australia, the Rutaceae is unconventional in having a higher net diversification rate of genera in the south-east than in the south-west from the Eocene–Oligocene (~34 million years ago) extinction pulse until the mid-Miocene (Nge et al. 2020). Resolution of the backbone polytomy would enable the further testing of hypotheses relating to the timing of this vicariance event in the Australian Rutaceae and offer insight into the drivers of generic diversification, and, specifically, whether the diversification of major lineages (i.e. our Clades 1–4) was influenced by such an event. It could also present an opportunity to provide a more robust timing for the split of Myrtopsis in New Caledonia.
Future classification of Philotheca
The recovery of Philotheca as polyphyletic in our analyses is consistent with previous work (Bayly et al. 2013; Appelhans et al. 2021; Duretto et al. 2023), and here we have provided further clarification of this taxonomic issue. Prior to the current study, the best estimate of relationships in Philotheca was provided by Duretto et al. (2023). Our analyses and that of Duretto et al. (2023) both suggest that Philotheca section Corynonema and Philotheca section Cyanochlamys are phylogenetically distant from the type of the genus (P. salsolifolia). Undoubtedly, they should be placed in separate genera from Philotheca and each other. However, in the case of P. section Cyanochlamys, contrasting support for monophyly (ptDNA; Fig. 3) v. paraphyly (nrDNA; see the Supplementary ‘Methods and results’ section) in our analyses raises questions about the appropriate taxonomic status of that group relative to the related, monotypic genus Muiriantha.
Within Clade 1, we have identified three ptDNA lineages of Philotheca species that render Philotheca paraphyletic with respect to Drummondita and Geleznowia, namely the following: (1) Philotheca section Erionema, supported as monophyletic and sister to the rest of the clade; (2) Philotheca s.str., not resolved as monophyletic but strongly placed in a clade with Drummondita; and (3) Philotheca ‘Nigrostipulae’, supported as monophyletic and sister to Geleznowia. Taxonomic interpretation of these relationships could include ‘lumping’ (e.g. placing all of Clade 1 in a single genus, Philotheca) or ‘splitting’ to various extents. Given that many of the species in this clade have been sampled only for a small number of plastid markers (Fig. 4), and that nrDNA sampling here (see the Supplementary ‘Methods and results’ section) and by Duretto et al. (2023) is very limited and does not resolve distinct nrDNA groups corresponding to Philotheca s.str. and Philotheca ‘Nigrostipulae’, some aspects of relationships in the group remain uncertain.
Given limitations of the results presented here, and the new questions they have raised, we consider that further investigation of the relationships of Philotheca is warranted before taxonomic changes are proposed. In particular, sequencing approaches that generate large amounts of data from the nuclear genome, such as using the MyBaits Expert Plant Angiosperms353 bait set (Arbor Bioscience, Ann Arbor, MI, USA; Johnson et al. 2019) for target-sequence capture (H. K. Orel, unpubl. data), could prove useful for elucidating unresolved relationships, because of the faster evolutionary rates of nuclear genes. Such data would also enable comparison with the ptDNA results presented here so as to evaluate the extent of cytonuclear discordance in this group.
Conclusions
This study has identified four major lineages of genera in the Eriostemon group with high support, significantly improving understanding of generic relationships in the Australasian Rutaceae (subfam. Zanthoxyloideae). Our analyses of full plastome sequences were unable to resolve the branching order of these major lineages; thus, they were treated as a polytomy and the true phylogenetic arrangement of the lineages remains unclear. Tests to clarify and identify the cause of this polytomy were inconclusive, but suggested greater support for topologies with the Philotheca–Drummondita–Geleznowia alliance (Clade 1) sister to the three other major lineages. This result also indicated that a bifurcating resolution of the polytomy is probably possible with a dataset more appropriate for investigating this question. Although the methods that we used to investigate the polytomy ultimately did not result in its resolution, we feel that these approaches are likely to be more powerful when applied to nDNA sequence data, because they are largely aimed at detecting and dealing with discordant phylogenetic signal across independently evolving loci or genes. Consequently, future phylogenomic studies of the Eriostemon group using sequence data from the nuclear genome hold much promise for improving phylogenetic resolution of the polytomy. Finally, our results reinforced previous findings that Philotheca is polyphyletic, and raised new questions regarding current sectional limits and monophyly that require further investigation before taxonomic changes can be made.
Note added in proof
In the period of time between acceptance and publication of this paper, a study on Geleznowia has been published that has increased the number of species in Geleznowia from two to seven (Anderson et al. 2023).
Data availability
GenBank accession numbers for the sequences used in this study can be found in Table 1. The datasets generated and analysed during the current study are available in the Figshare repository (https://doi.org/10.26188/21761735). N.B.: as several taxonomic revisions were made during this study, sequences in the Figshare datasets may not incorporate the taxonomic changes of Duretto et al. (2020, 2023) and Telford and Bruhl (2020), which are incorporated in this paper. Specifically, sequences of Leionema beckleri (PIF33439), Cyanothamnus anemonifolius (MJB2562), Chorilaena anceps (BJM475), Chorilaena euphemiae (BJM424) and Phebalium multiflorum (RB1280) may be respectively named Leionema elatius, Boronia anemonifolia, Rhadinothamnus anceps, Rhadinothamnus euphemiae and Microcybe multiflora.
Conflicts of interest
M. J. Bayly is an editor for Australian Systematic Botany. Despite this relationship, he did not at any stage have editor-level access to this manuscript while in peer review, as is the standard practice when handling manuscripts submitted by an editor to this journal. Australian Systematic Botany encourages its editors to publish in the journal and has protocols that keep editors separate from the decision-making processes for their manuscripts. The authors have no conflicts of interests to declare that are relevant to the content of this article.
Declaration of funding
This research was supported by an Early Career Researcher Grant from The University of Melbourne. It also used collections made under previous grants from the Australian Research Council (grant LP0776737) and Australian Biological Resources Study (grant PD208-02). H. K. Orel is supported by an Australian Government Research Training Program scholarship, and a Sophie Ducker scholarship from the University of Melbourne Botany Foundation.
Author contributions
M. J. Bayly and H. K. Orel conceived the ideas and experimental design for this study. P. I. Forster and M. J. Bayly contributed samples. W. C. Neal, T. G. B. McLay and M. J. Bayly performed laboratory work to generate the sequence data. H. K. Orel assembled the sequence data and conducted all analyses; T. G. B. McLay assisted with topology testing and preliminary analyses. Results were interpreted by H. K. Orel, T. G. B. McLay and M. J. Bayly. H. K. Orel led the writing, with input from all other authors.
Acknowledgements
The authors thank Bryan Mole, Adrienne Markey, Jeremy Bruhl, Daniel Ohlsen, Mike Mathieson, Gillian Brown, Peter Wilson and Adele Gibbs for assistance with fieldwork or provision and use of samples; and Freya Berwick, Katie Roland, Miranda Boyle (all intern students), Jessie Knott, Erin Batty, Gareth Holmes, Rachael Fowler, and Stephen Wilcox for assistance with laboratory work or DNA sequencing. Collecting permits were provided by the former Victorian Department of Sustainability and Environment, NSW National Parks and Wildlife Service, South Australian Department of Environment and Heritage, the former Western Australian Department of Environment and Conservation, and the former Queensland Environmental Protection Agency.
References
Anderson BM, Binks RM, Byrne M, Crawford AD, Shepherd KA (2023) Using RADseq to resolve species boundaries in a morphologically complex group of yellow-flowered shrubs (Geleznowia, Rutaceae). Australian Systematic Botany 36(4), 277-311.
| Crossref | Google Scholar |
Appelhans MS, Bayly MJ, Heslewood MM, Groppo M, Verboom GA, Forster PI, Kallunki JA, Duretto MF (2021) A new subfamily classification of the Citrus family (Rutaceae) based on six nuclear and plastid markers. Taxon 70, 1035-1061.
| Crossref | Google Scholar |
Armstrong JA (1979) Biotic pollination mechanisms in the Australian flora – a review. New Zealand Journal of Botany 17, 467-508.
| Crossref | Google Scholar |
Armstrong JA (1987) Pollination syndromes as generic determinants. Australian Systematic Botany Society Newsletter 53, 54-59.
| Google Scholar |
Armstrong JA (1991) Studies on pollination and systematics in the Australian Rutaceae. PhD thesis, University of New South Wales, Sydney, NSW, Australia. Available at http://hdl.handle.net/1959.4/59714
Baker WJ, Bailey P, Barber V, Barker A, Bellot S, Bishop D, Botigué LR, Brewer G, Carruthers T, Clarkson JJ, Cook J (2022) A comprehensive phylogenomic platform for exploring the angiosperm tree of life. Systematic Biology 71, 301-319.
| Crossref | Google Scholar |
Bardon L, Sothers C, Prance GT, Malé P-JG, Xi Z, Davis CC, Murienne J, García-Villacorta R, Coissac E, Lavergne S, Chave J (2016) Unraveling the biogeographical history of Chrysobalanaceae from plastid genomes. American Journal of Botany 103, 1089-1102.
| Crossref | Google Scholar |
Barrett RA, Bayly MJ, Duretto MF, Forster PI, Ladiges PY, Cantrill DJ (2014) A chloroplast phylogeny of Zieria (Rutaceae) in Australia and New Caledonia shows widespread incongruence with species-level taxonomy. Australian Systematic Botany 27, 427-449.
| Crossref | Google Scholar |
Batty EL, Holmes GD, Murphy DJ, Forster PI, Neal WC, Bayly MJ (2022) Phylogeny, classification and biogeography of Philotheca sect. Erionema (Rutaceae) based on nrDNA sequences. Australian Systematic Botany 35, 326-338.
| Crossref | Google Scholar |
Bayly MJ (2001) A cladistic and biogeographic analysis of Philotheca (Rutaceae) and allied genera. PhD thesis, The University of Melbourne, Melbourne, Vic., Australia. Available at http://hdl.handle.net/11343/37369
Bayly MJ, Holmes GD, Forster PI, Cantrill DJ, Ladiges PY (2013) Major clades of Australasian Rutoideae (Rutaceae) based on rbcL and atpB sequences. PLoS One 8, e72493.
| Crossref | Google Scholar |
Bayly MJ, Holmes GD, Forster PI, Munzinger J, Cantrill DJ, Ladiges PY (2016) Phylogeny, classification and biogeography of Halfordia (Rutaceae) in Australia and New Caledonia. Plant Systematics and Evolution 302, 1457-1470.
| Crossref | Google Scholar |
Broadhurst LM, Tan BH (2001) Floral biology of the Western Australian endemic ‘yellow bells’, Geleznowia verrucosa Turcz (Rutaceae). Journal of the Royal Society of Western Australia 84, 83-89.
| Google Scholar |
Buckler ES, Holtsford TP (1996) Zea systematics: ribosomal ITS evidence. Molecular Biology and Evolution 13, 612-622.
| Crossref | Google Scholar |
Clowes C, Fowler RM, Fahey PS, Kellermann J, Brown GK, Bayly MJ (2022) Big trees of small baskets: phylogeny of the Australian genus Spyridium (Rhamnaceae: Pomaderreae), focusing on biogeographic patterns and species circumscriptions. Australian Systematic Botany 35, 95-119.
| Crossref | Google Scholar |
Crisp MD, Cook LG (2007) A congruent molecular signature of vicariance across multiple plant lineages. Molecular Phylogenetics and Evolution 43, 1106-1117.
| Crossref | Google Scholar |
da Silva MFGF, Gottlieb OR, Ehrendorfer F (1988) Chemosystematics of the Rutaceae: suggestions for a more natural taxonomy and evolutionary interpretation of the family. Plant Systematics and Evolution 161, 97-134.
| Crossref | Google Scholar |
Doyle JJ (1997) Trees within trees: genes and species, molecules and morphology. Systematic Biology 46, 537-553.
| Crossref | Google Scholar |
Doyle JJ (2021) Defining coalescent genes: theory meets practice in organelle phylogenomics. Systematic Biology 71, 476-489.
| Crossref | Google Scholar |
Duchêne DA, Bragg JG, Duchêne S, Neaves LE, Potter S, Moritz C, Johnson RN, Ho SYW, Eldridge MDB (2018) Analysis of phylogenomic tree space resolves relationships among marsupial families. Systematic Biology 67, 400-412.
| Crossref | Google Scholar |
Duretto MF, Heslewood MM, Bayly MJ (2020) Boronia (Rutaceae) is polyphyletic: reinstating Cyanothamnus and the problems associated with inappropriately defined outgroups. Taxon 69, 481-499.
| Crossref | Google Scholar |
Duretto MF, Heslewood MM, Bayly MJ (2023) Generic and infrageneric limits of Phebalium and its allies (Rutaceae: Zanthoxyloideae). Australian Systematic Botany 36, 107-142.
| Crossref | Google Scholar |
Edwards SV (2009) Is a new and general theory of molecular systematics emerging. Evolution 63, 1-19.
| Crossref | Google Scholar |
Foster CSP, Henwood MJ, Ho SYW (2018) Plastome sequences and exploration of tree-space help to resolve the phylogeny of riceflowers (Thymelaeaceae: Pimelea). Molecular Phylogenetics and Evolution 127, 156-167.
| Crossref | Google Scholar |
French PA, Brown GK, Bayly MJ (2016) Incongruent patterns of nuclear and chloroplast variation in Correa (Rutaceae): introgression and biogeography in south-eastern Australia. Plant Systematics and Evolution 302, 447-468.
| Crossref | Google Scholar |
Gonçalves DJP, Simpson BB, Ortiz EM, Shimizu GH, Jansen RK (2019) Incongruence between gene trees and species trees and phylogenetic signal variation in plastid genes. Molecular Phylogenetics and Evolution 138, 219-232.
| Crossref | Google Scholar |
Gonçalves DJP, Jansen RK, Ruhlman TA, Mandel JR (2020) Under the rug: abandoning persistent misconceptions that obfuscate organelle evolution. Molecular Phylogenetics and Evolution 151, 106903.
| Crossref | Google Scholar |
Grandcolas P (2017) Ten false ideas about New Caledonia biogeography. Cladistics 33, 481-487.
| Crossref | Google Scholar |
Groppo M, Pirani JR, Salatino MLF, Blanco SR, Kallunki JA (2008) Phylogeny of Rutaceae based on two noncoding regions from cpDNA. American Journal of Botany 95, 985-1005.
| Crossref | Google Scholar |
Groppo M, Kallunki JA, Pirani JR, Antonelli A (2012) Chilean Pitavia more closely related to Oceania and Old World Rutaceae than to Neotropical groups: evidence from two cpDNA non-coding regions, with a new subfamilial classification of the family. PhytoKeys 19, 9-29.
| Crossref | Google Scholar |
Guindon S, Dufayard J-F, Lefort V, Anisimova M, Hordijk W, Gascuel O (2010) New algorithms and methods to estimate maximum-likelihood phylogenies: assessing the performance of PhyML 3.0. Systematic Biology 59, 307-321.
| Crossref | Google Scholar |
Hammer TA, Zhong X, Colas des Francs‐Small C, Nevill PG, Small ID, Thiele KR (2019) Resolving intergeneric relationships in the aervoid clade and the backbone of Ptilotus (Amaranthaceae): evidence from whole plastid genomes and morphology. Taxon 68, 297-314.
| Crossref | Google Scholar |
Hartley TG (1995) A new combination in Boronella (Rutaceae) and a view on relationships of the genus. Adansonia 17, 107-111.
| Google Scholar |
Hartley TG (2001) Morphology and biogeography in Australasian–Malesian Rutaceae. Malayan Nature Journal 55, 197-220.
| Google Scholar |
Hartley TG (2003) Neoschmidia, a new genus of Rutaceae from New Caledonia. Adansonia 25, 7-12.
| Google Scholar |
Hoang DT, Chernomor O, von Haeseler A, Minh BQ, Vinh LS (2018) UFBoot2: improving the ultrafast bootstrap approximation. Molecular Biology and Evolution 35, 518-522.
| Crossref | Google Scholar |
Hou N, Wang G, Feng SJ, Wei AZ (2018) The complete chloroplast genome of an aromatic Chinese pepper (Zanthoxylum simulans). Mitochondrial DNA Part B 3, 26-27.
| Crossref | Google Scholar |
Huson DH, Bryant D (2006) Application of phylogenetic networks in evolutionary studies. Molecular Biology and Evolution 23, 254-267.
| Crossref | Google Scholar |
Jin J-J, Yu W-B, Yang J-B, Song Y, DePamphilis CW, Yi T-S, Li D-Z (2020) GetOrganelle: a fast and versatile toolkit for accurate de novo assembly of organelle genomes. Genome Biology 21, 241.
| Crossref | Google Scholar |
Johnson MG, Pokorny L, Dodsworth S, Botigué LR, Cowan RS, Devault A, Eiserhardt WL, Epitawalage N, Forest F, Kim JT, Leebens-Mack JH, Leitch IJ, Maurin O, Soltis DE, Soltis PS, Wong GK, Baker WJ, Wickett NJ (2019) A universal probe set for targeted sequencing of 353 nuclear genes from any flowering plant designed using k-medoids clustering. Systematic Biology 68, 594-606.
| Crossref | Google Scholar |
Jombart T, Kendall M, Almagro-Garcia J, Colijn C (2017) treespace: statistical exploration of landscapes of phylogenetic trees. Molecular Ecology Resources 17, 1385-1392.
| Crossref | Google Scholar |
Joyce EM, Appelhans MS, Buerki S, et al. (2023) Phylogenomic analyses of Sapindales support new family relationships, rapid Mid-Cretaceous Hothouse diversification, and heterogeneous histories of gene duplication. Frontiers in Plant Science 14, 1063174.
| Crossref | Google Scholar |
Kalyaanamoorthy S, Minh BQ, Wong TKF, von Haeseler A, Jermiin LS (2017) ModelFinder: fast model selection for accurate phylogenetic estimates. Nature Methods 14, 587-589.
| Crossref | Google Scholar |
Katoh K, Standley DM (2013) MAFFT multiple sequence alignment software version 7: improvements in performance and usability. Molecular Biology and Evolution 30, 772-780.
| Crossref | Google Scholar |
Katoh K, Misawa K, Kuma K-i, Miyata T (2002) MAFFT: a novel method for rapid multiple sequence alignment based on fast Fourier transform. Nucleic Acids Research 30, 3059-3066.
| Crossref | Google Scholar |
Kishino H, Hasegawa M (1989) Evaluation of the maximum likelihood estimate of the evolutionary tree topologies from DNA sequence data, and the branching order in hominoidea. Journal of Molecular Evolution 29, 170-179.
| Crossref | Google Scholar |
Kishino H, Miyata T, Hasegawa M (1990) Maximum likelihood inference of protein phylogeny and the origin of chloroplasts. Journal of Molecular Evolution 31, 151-160.
| Crossref | Google Scholar |
Ladiges PY, Cantrill D (2007) New Caledonia-Australian connections: biogeographic patterns and geology. Australian Systematic Botany 20, 383-389.
| Crossref | Google Scholar |
Ladiges PY, Bayly MJ, Nelson G (2012) Searching for ancestral areas and artifactual centers of origin in biogeography: with comment on east–west patterns across southern Australia. Systematic Biology 61, 703-708.
| Crossref | Google Scholar |
Lin GN, Zhang C, Xu D (2011) Polytomy identification in microbial phylogenetic reconstruction. BMC Systems Biology 5, S2.
| Crossref | Google Scholar |
Minh BQ, Hahn MW, Lanfear R (2020a) New methods to calculate concordance factors for phylogenomic datasets. Molecular Biology and Evolution 37, 2727-2733.
| Crossref | Google Scholar |
Minh BQ, Schmidt HA, Chernomor O, Schrempf D, Woodhams MD, von Haeseler A, Lanfear R (2020b) IQ-TREE 2: new models and efficient methods for phylogenetic inference in the genomic era. Molecular Biology and Evolution 37, 1530-1534.
| Crossref | Google Scholar |
Mole BJ, Udovicic F, Ladiges PY, Duretto MF (2004) Molecular phylogeny of Phebalium (Rutaceae: Boronieae) and related genera based on the nrDNA regions ITS 1 + 2. Plant Systematics and Evolution 249, 197-212.
| Crossref | Google Scholar |
Mueller F (1869) ‘Fragmenta phytographiæ Australiæ. Vol. 7.’ (Auctoritate Guberni Coloniæ Victoriæ, Ex Officina Joannis Ferres: Melbourne, Australia) Available at https://www.biodiversitylibrary.org/item/7224
Nattier R, Pellens R, Robillard T, Jourdan H, Legendre F, Caesar M, Nel A, Grandcolas P (2017) Updating the phylogenetic dating of New Caledonian biodiversity with a meta-analysis of the available evidence. Scientific Reports 7, 3705.
| Crossref | Google Scholar |
Neal WC, James EA, Bayly MJ (2019) Phylogeography, classification and conservation of pink zieria (Zieria veronicea; Rutaceae): influence of changes in climate, geology and sea level in south-eastern Australia. Plant Systematics and Evolution 305, 503-520.
| Crossref | Google Scholar |
Nge FJ, Biffin E, Thiele KR, Waycott M (2020) Extinction pulse at Eocene–Oligocene boundary drives diversification dynamics of two Australian temperate floras. Proceedings of the Royal Society of London – B. Biological Sciences 287, 20192546.
| Crossref | Google Scholar |
Othman RNA, Jordan GJ, Worth JRP, Steane DA, Duretto MF (2010) Phylogeny and infrageneric classification of Correa Andrews (Rutaceae) on the basis of nuclear and chloroplast DNA. Plant Systematics and Evolution 288, 127-138.
| Crossref | Google Scholar |
Palumbi SR, Cipriano F, Hare MP (2001) Predicting nuclear gene coalescence from mitochondrial data: the three-times rule. Evolution 55, 859-868.
| Crossref | Google Scholar |
Penny D, Hendy MD (1985) The use of tree comparison metrics. Systematic Zoology 34, 75-82.
| Crossref | Google Scholar |
Rambaut A, Drummond AJ, Xie D, Baele G, Suchard MA (2018) Posterior summarization in Bayesian phylogenetics using Tracer 1.7. Systematic Biology 67, 901-904.
| Crossref | Google Scholar |
Revell LJ (2012) phytools: an R package for phylogenetic comparative biology (and other things). Methods in Ecology and Evolution 3, 217-223.
| Crossref | Google Scholar |
Rieseberg LH, Soltis DE (1991) Phylogenetic consequences of cytoplasmic gene flow in plants. Evolutionary Trends in Plants 5, 65-84.
| Google Scholar |
Ronquist F, Teslenko M, van der Mark P, Ayres DL, Darling A, Höhna S, Larget B, Liu L, Suchard MA, Huelsenbeck JP (2012) MrBayes 3.2: efficient Bayesian phylogenetic inference and model choice across a large model space. Systematic Biology 61, 539-542.
| Crossref | Google Scholar |
Sang T, Crawford DJ, Stuessy TF (1997) Chloroplast DNA phylogeny, reticulate evolution, and biogeography of Paeonia (Paeoniaceae). American Journal of Botany 84, 1120-1136.
| Crossref | Google Scholar |
Sayyari E, Mirarab S (2018) Testing for polytomies in phylogenetic species trees using quartet frequencies. Genes 9, 132.
| Crossref | Google Scholar |
Schuster TM, Setaro SD, Tibbits JFG, Batty EL, Fowler RM, McLay TGB, Wilcox S, Ades PK, Bayly MJ (2018) Chloroplast variation is incongruent with classification of the Australian bloodwood eucalypts (genus Corymbia, family Myrtaceae). PLoS One 13, e0195034.
| Crossref | Google Scholar |
Shepherd LD, McLay TGB (2011) Two micro-scale protocols for the isolation of DNA from polysaccharide-rich plant tissue. Journal of Plant Research 124, 311-314.
| Crossref | Google Scholar |
Shimodaira H (2002) An approximately unbiased test of phylogenetic tree selection. Systematic Biology 51, 492-508.
| Crossref | Google Scholar |
Shimodaira H, Hasegawa M (1999) Multiple comparisons of log-likelihoods with applications to phylogenetic inference. Molecular Biology and Evolution 16, 1114-1116.
| Crossref | Google Scholar |
Slowinski JB (2001) Molecular Polytomies. Molecular Phylogenetics and Evolution 19, 114-120.
| Crossref | Google Scholar |
Smith MR (2022) Robust Analysis of Phylogenetic Tree Space. Systematic Biology 71, 1255-1270.
| Crossref | Google Scholar |
Smith-White S (1954) Chromosome numbers in the Boronieae (Rutaceae) and their bearing on the evolutionary development of the tribe in the Australian flora. Australian Journal of Botany 2, 287-303.
| Crossref | Google Scholar |
Stace HM, Armstrong JA (1992) New chromosome numbers for Rutaceae. Australian Systematic Botany 5, 501-505.
| Crossref | Google Scholar |
Stamatakis A (2014) RAxML version 8: a tool for phylogenetic analysis and post-analysis of large phylogenies. Bioinformatics 30, 1312-1313.
| Crossref | Google Scholar |
Strimmer K, Rambaut A (2002) Inferring confidence sets of possibly misspecified gene trees. Proceedings of the Royal Society of London – B. Biological Sciences 269, 137-142.
| Crossref | Google Scholar |
Strimmer K, von Haeseler A (1997) Likelihood-mapping: a simple method to visualize phylogenetic content of a sequence alignment. Proceedings of the National Academy of Sciences 94, 6815-6819.
| Crossref | Google Scholar |
Tate JA, Simpson BB (2003) Paraphyly of Tarasa (Malvaceae) and diverse origins of the polyploid species. Systematic Botany 28, 723-737.
| Crossref | Google Scholar |
Telford IRH, Bruhl JJ (2020) Morphological data indicate the subspecies of Leionema elatius (Rutaceae) are not conspecific. Telopea 23, 197-203.
| Crossref | Google Scholar |
Tibshirani R, Walther G, Hastie T (2001) Estimating the number of clusters in a data set via the gap statistic. Journal of the Royal Statistical Society – B. Statistical Methodology 63, 411-423.
| Crossref | Google Scholar |
Tillich M, Lehwark P, Pellizzer T, Ulbricht-Jones ES, Fischer A, Bock R, Greiner S (2017) GeSeq – versatile and accurate annotation of organelle genomes. Nucleic Acids Research 45, W6-W11.
| Crossref | Google Scholar |
Toews DPL, Brelsford A (2012) The biogeography of mitochondrial and nuclear discordance in animals. Molecular Ecology 21, 3907-3930.
| Crossref | Google Scholar |
Tsitrone A, Kirkpatrick M, Levin DA (2003) A model for chloroplast capture. Evolution 57, 1776-1782.
| Crossref | Google Scholar |
Whitfield JB, Lockhart PJ (2007) Deciphering ancient rapid radiations. Trends in Ecology & Evolution 22, 258-265.
| Crossref | Google Scholar |
Williams AV, Miller JT, Small I, Nevill PG, Boykin LM (2016) Integration of complete chloroplast genome sequences with small amplicon datasets improves phylogenetic resolution in Acacia. Molecular Phylogenetics and Evolution 96, 1-8.
| Crossref | Google Scholar |
Wilson PG (1970) A taxonomic revision of the genera Crowea, Eriostemon and Phebalium (Rutaceae). Nuytsia 1, 3-155.
| Crossref | Google Scholar |
Wilson PG (1971) Taxonomic notes on the family Rutaceae, principally of Western Australia. Nuytsia 1, 197-207.
| Crossref | Google Scholar |
Wilson PG (1998a) A taxonomic review of the genera Eriostemon and Philotheca (Rutaceae: Boronieae). Nuytsia 12, 239-265.
| Google Scholar |
Wilson PG (1998b) New species and nomenclatural changes in Phebalium and related genera (Rutaceae). Nuytsia 12, 267-288.
| Google Scholar |
Wolfe KH, Li WH, Sharp PM (1987) Rates of nucleotide substitution vary greatly among plant mitochondrial, chloroplast, and nuclear DNAs. Proceedings of the National Academy of Sciences 84, 9054-9058.
| Crossref | Google Scholar |
Zeng L, Zhang Q, Sun R, Kong H, Zhang N, Ma H (2014) Resolution of deep angiosperm phylogeny using conserved nuclear genes and estimates of early divergence times. Nature Communications 5, 4956.
| Crossref | Google Scholar |
Zhang C, Rabiee M, Sayyari E, Mirarab S (2018) ASTRAL-III: polynomial time species tree reconstruction from partially resolved gene trees. BMC Bioinformatics 19, 153.
| Crossref | Google Scholar |