A simple 3D printed microfluidic device for point-of-care analysis of urinary uric acid
Kolsoum Dalvand A , Alireza Ghiasvand
A Department of Chemistry, Lorestan University, Khoramabad, Iran.
B Australian Centre for Research on Separation Science (ACROSS), School of Natural Sciences, University of Tasmania, Hobart, Tas. 7001, Australia.
Australian Journal of Chemistry 76(2) 74-80 https://doi.org/10.1071/CH22180
Submitted: 14 August 2022 Accepted: 8 December 2022 Published: 10 February 2023
© 2023 The Author(s) (or their employer(s)). Published by CSIRO Publishing. This is an open access article distributed under the Creative Commons Attribution-NonCommercial-NoDerivatives 4.0 International License (CC BY-NC-ND)
Abstract
Point-of-care testing (POCT) technology allows scientists to monitor and diagnose diseases at the patient site, much faster than classical lab-based methods. Herein, a rapid, simple, and sensitive 3D printed microfluidic device integrated with smartphone-based on-chip detection is described for POCT quantification of urinary uric acid. The device includes two circular inputs each connected to a microliter-scale chamber, separated by an integrated porous membrane, located between the sample and reagent chambers. The microfluidic device was fabricated from a transparent photopolymer using a 3D printer, in a single run. The concentration of uric acid was determined based on a chromogenic reaction in which ferrous ion, produced via the reduction of ferric ion by the analyte, complexed with 1,10-phenanthroline, and the color was recorded by a smartphone. Response surface methodology including a central composed design was utilized to evaluate the experimental parameters and subsequent introduction of a multivariate model to describe the experimental conditions. Under the optimum conditions, the calibration curve was linear over the concentration range of 30–600 mg L−1. The limit of detection was determined to be 10.5 mg L−1. The microfluidic device was successfully utilized for the recovery and quantification of uric acid in the urine, with recoveries ranging from 91.7 to 99.7%.
Keywords: 1,10-phenanthroline, 3D printing, colorimetric detection, microfluidic, point-of-care testing, smartphone-based on-chip detection, uric acid, urine.
Introduction
Uric acid (7,9-dihydro-1H-purine-2,6,8(3H)-trione), composed of welded rings of imidazole and pyrimidine, is the end breakdown product of dietary or endogenous purines.[1] Uric acid concentration in urine and blood serum for healthy persons is within the ranges of 250–750 mg L−1 and 15–80, respectively. Diseases such as leukemia, hyperuricemia, Lesch–Nyhan syndrome, gout, lymphoma, and renal failure cause high levels of serum uric acid (SUA) and consequently high levels of uric acid in the urine.[2] Moreover, high uric acid levels can be associated with diabetes and high cholesterol.[3] Accordingly, monitoring uric acid levels in blood and urine is a very useful marker for disease diagnosis. Various analytical methods have been reported for the measurement of uric acid in human urine and blood, which can be classified into enzymatic and non-enzymatic methods. Uricase catalysts fit in the enzymatic methods and generate carbon dioxide, allantoin, and hydrogen peroxide from uric acid. Uric acid concentration is then determined by measuring hydrogen peroxide.[4] In the non-enzymatic procedure, uric acid is determined directly, for example using electrophoresis,[5] liquid chromatography,[6] spectrofluorimetry,[7] or electrochemical methods.[8] Although these procedures have acceptable sensitivity and selectivity for the measurement of uric acid, they are lab-based techniques, time-consuming, and generally need large sample volumes and expensive instrumentations. To address this challenge, many efforts have been dedicated to developing simple and low-cost portable devices for point-of-care testing of clinical markers, like uric acid.[9] Micro total analysis systems or lab-on-a-chip systems have been introduced in recent years to reduce analysis time, labor, and cost of chemical analysis of biological, clinical, and environmental samples, particularly based on microfluidic chips.[10–12] Microfluidic technologies have well proven their reliability and applicability for the preparation of point-of-care testing (POCT) devices for disease screening and on-site diagnosis, due to their useful features of high throughput, high speed, low-cost, low sample consumption, and simplicity.[13]
So far, different microfluidic systems have been used for diagnosis and monitoring diseases such as diabetes, cardiovascular disease, renal disease, and cancer.[14] Rossini et al. employed a paper-based microfluidic analytical device for the simultaneous quantification of uric acid and creatinine in urine.[15] Chen et al. used a 3D-printed paper-based microfluidic device and used it for bienzyme colorimetric determination of uric acid and glucose in human serum.[16] However, traditional technologies to produce microfluidic devices such as soft lithography, laser ablation, micro-machining, hot embossing, and injection molding are expensive, require skilled operators, and sophisticated instruments. They have also limited options for the preparation of biocompatible systems.[17,18]
3D printing technology is a promising alternative to address these limitations and can be used for low-cost and fast fabrication of micro/mesofluidic devices with complex geometry with high repeatability.[19] Among different commercially available 3D printing technologies, PolyJet is preferred for the fabrication of small objects with more details and fine surface finishing like microfluidic devices that need higher structural resolution.[20] In PolyJet printing, liquid photopolymers are jetted through linearly arranged nozzles and the resultant microdroplets sprayed onto the build surface, where the materials are solidified by being irradiated using ultraviolet light. These printers have a high throughput but offer a limited selection of photopolymer build materials.[21]
This research reports and evaluates a low-cost and simple microfluidic device for POCT quantification of uric acid in urine. The 3D printed microfluidic device was fabricated by a transparent photopolymer by a PolyJet printer in a single run and employed for indirect image-based colorimetric determination of uric acid using a smartphone. The influential parameters on the performance of the smartphone-based microfluidic system were optimized via a response surface methodology (RSM) including central composite design (CCD).
Experimental section
Chemicals and apparatus
Analytical reagent grade uric acid, 1,10-phenanthroline, Fe(NO3)3.9H2O and all other acids, bases, and salts were purchased from Merck (Darmstadt, Germany). A standard stock solution (1000 µg mL−1) of uric acid was prepared in 0.05 mol L−1 sodium hydroxide solution. The working standard solutions were made by diluting the corresponding stock solution with 0.01 M phosphate-buffered saline solution (pH ~7.4). PolyJet water-soluble support material (SUP7070) and build material (VeroClear™ RGD810, transparent photopolymer) were provided by Stratasys (Eden Prairie, MN, USA). An Objet Eden260VS 3D printer from Stratasys was used for 3D printing of the chips with a resolution of 600 × 600 × 1600 dpi and a 16-μm layer thickness. An Orbital Shaker SO1 from Stuart Scientific (Staffordshire, UK) was utilized to remove the support material from the chips by washing with 0.2% NaOH solution. A Shimadzu UV-1650 PC spectrophotometer was used to record absorption spectra of uric acid and Fe2+/1,10-orthophenanthroline complex.
Design and fabrication of microfluidic chip
The microfluidic device with an integrated membrane between the chambers was printed using the PolyJet printer in a single run as described previously.[21] Briefly, the chip was first designed by Version 2016 AutoCAD software (Autodesk Inc., CA, USA). The CAD files were then transformed to STL format and sliced into G-codes (ready to print) by KISSlicer PRO software. The chip contains two 35-μL chambers interconnected by a porous membrane. Each chamber has a circular hole as input. The sample chamber was designed in a rectangular shape, with a triangular channel reagent chamber.
The dimensions of the microfluidic device were 40 × 20 × 1.4 mm. After printing, surplus layers of the support were first removed using a knife, and the chips were rinsed several times with 0.2% NaOH solution. Then, they were soaked in 0.2% NaOH solution and shaken for 24 h, for the complete removal of the support material and cleaning of the chambers. Finally, the microfluidic devices were dried at ambient temperature on a clean aluminum foil and kept in sealed plastic bags. To avoid the adsorption of analytes onto chambers and the membrane, each microfluidic device was used in a single test.
Results and discussion
Smartphone-based colorimetric microfluidic determination of uric acid
Uric acid is a strong electron donor (reducing agent), and this characteristic was exploited to develop a strategy for its determination via a smartphone-based colorimetric microfluidic method.[22] For this purpose, first, ferric ion (Fe3+) was reduced by uric acid and converted to ferrous ion (Fe2+). Then, ferrous ion was reacted with 1,10-phenanthroline to form ferroin (a 1:3 complex, [Fe(phen)3]2+) and immediately generated a pale-yellow color in the pH range 2–9. Ferroin is a suitable redox indicator and is widely applied in titrimetry, particularly cerimetric titration. Its color change is reversible and very fast. The color of Ferroin solutions is stable for several weeks at temperatures up to 60°C. The overall reaction of Fe3+ with uric acid in the presence of 1,10-phenanthroline is depicted in Fig. 1.
![]() |
For colorimetric determination of uric acid using the microfluidic device, 35 μL uric acid solution (sample) was injected into the rectangular chamber and then 35 µL of chromogenic reagent (Fe3+1,10-phenanthroline) mixture was injected into to the triangular chamber and left to equilibrate (room temperature, 12.5 min).
Fig. 2 shows the images of a microfluidic device before and after the injection of the chromogenic reagent and sample. The sample chamber was colorless at first but turned pale yellow as the chromogenic reagent began to penetrate the sample. After completing color development, the sample chamber turned red. Then, a smartphone was used to photograph the microfluidic devices at a distance of 10 cm. The processing of the obtained images was accomplished using Image J (https://imagej.nih.gov/ij). The recorded intensities were transformed to greyscale after subtracting the intensity of the background. Then the responses of the samples were compared to a standard calibration graph. The design that illustrates the whole set operating protocol for the smartphone-based colorimetric determination of urinary uric acid is shown in Supplementary Fig. S1 and the injection, color development, and data processing steps are depicted in Supplementary Fig. S2.
![]() |
Optimization of the smartphone-based colorimetric method using RSM-CCD
RSM uses a low-order polynomial equation in a predefined range of a set of independent variables. It is then analyzed to determine the optimal values of the variables in order to obtain the best response.[23] Multivariate optimization is faster than a one-at-a-time strategy and requires fewer experimental runs. It is also able to clarify the interactions between the factors. Therefore, response surface methodology was used to identify the parameters that had the greatest influence on the chromogenic reaction, including reaction time, the concentration of ferric ion, and concentration of 1,10-phenanthroline. CCD, as a response surface strategy, combines a two-level factorial design with a star design and center points.[24] The number of experimental runs (N) for a CCD with k factors and Cp center points is defined by:

Three factors were defined as reaction time (min), concentrations of 1,10-phenanthroli (mol L−1), and concentrations of Fe3+ (mol L−1), at five center points (Supplementary Table S1). This means that 20 test runs had to be performed. The experimental matrix was designed using Minitab 19 statistical software (Minitab Inc., State College, PA, USA). The CCD experimental matrix and the obtained responses are given in Supplementary Table S2. Color intensity, recorded by the smartphone, was selected as the response.
A second-order polynomial equation has resulted from the multiple linear regression (Eqn 2). It explains the relationship between color intensity and the experimental factors as:
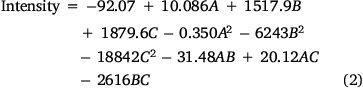
A two-way ANOVA was employed to compare the differences in the responses. The ANOVA was performed using Fisher statistical analysis and the results are shown in Supplementary Table S3. Based on the results, all studied variables had significant effects on the response (P < 0.05). A P-value of less than 0.05 indicates a parameter that is statistically significant, at 95% confidence level. The ‘Lack of Fit’ for the F-value is 0.682, which demonstrates it isn’t significant relative to the pure error, confirming the validity of the second-order polynomial model. Furthermore, the high coefficient of determination R2 (99.83%), adjusted R2 (99.61%), and predicted R2 (99.32%) show an acceptable correlation between the experimental variables and the selected model.[25] In addition, the response surface plots (Fig. 3a) were used to optimize the variables and evaluate the interactive effects of the independent factors, while the other factors were kept constant at their optimal values. As the results show, the optimum values of reaction time, the concentration of Fe3+, and the concentration of 1,10-phenanthroline were predicted 12.5 (min), 0.05 (mol L−1), and 0.08 (mol L−1), respectively. Fig. 3a also shows that the color intensity increases with reaction time to 12.5 min and then decreases. On the other hand, raising of Fe3+ and phenanthroline concentrations leads to an increase in color intensity with reaction time. The contour plots are presented in Fig. 3b, showing that there is no direct linear relationship between the selected independent variables.
The interaction between the parameters is shown in the plot interaction diagram (Supplementary Fig. S3). Non-parallel lines indicate the interaction between parameters. The diagram shows there are interactions between Time/Cphen and Time/CFe3+, but there is no interaction between Cphen/CFe3+.
Analytical figures of merit and real sample
The smartphone-based colorimetric method proposed in this research was evaluated by obtaining the analytical figures of merit, including, the limit of detection (LOD), relative standard deviation (RSD), and linear dynamic range (LDR). The calibration graph was linear over the range of 30–600 mg L−1 (R2 = 0.992). The LOD (three times the blank’s standard deviation, 3σ) was 10.5 mg L−1. RSD for six replicated analyzes of a sample containing 250 mg L−1 of uric acid was found to be 7.9%. The calibration graph and color change for different concentrations of uric acid obtained while generating the calibration graph are presented in Supplementary Figs S4 and S5, respectively.
To assess the applicability of the developed method for real samples, it was utilized for the measurement of uric acid in three human urine samples, collected from a local Pathobiology Laboratory (Khoramabad, Iran), in accordance with relevant guidelines and regulations. To ensure the accuracy and reliability of the obtained results, all samples were also fortified with a standard solution at three levels (close to low-quality control (LQC), medium quality control (MQC), and high-quality control (HQC)) according to the International Council for Harmonisation of Technical Requirements for Pharmaceuticals for Human Use recommendations[11,26] and subjected to the developed method in triplicate (Table 1). Recoveries were obtained in the range of 91.7–99.7%, demonstrating that the proposed microfluidic device and smartphone-based colorimetric procedure can be successfully applied for the determination of uric acid in urine samples.
![]() |
For further evaluation of the reliability and validity of the developed method, its analytical performance was compared with similar published studies on the determination of uric acid.[9,15,16,27] The results (Table 2) showed that the LOD of the proposed procedure is lower than other reported methods, except for the method that utilized chitosan to improve the analytical response of enzymatic bioassay via gel documentation.[9] The RSD is also comparable to the values reported for the listed procedures. These studies used photographic cameras,[27] benchtop spectrophotometers,[15] and benchtop scanners,[9] the former two of which impede the portability and point-of-care use of these systems. The only portable setup used in these studies (Table 2) is a Sigma-Aldrich ALT assay kit; however, the widespread use of this method would be limited by the high cost of the measurement kit (each kit sufficient for 100 colorimetric or fluorometric tests costs AU$668, as of 30 March 2022). In contrast, the procedure presented here uses a simple and low-cost colorimetric setup, integrated into a microfluidic chip, and is suitable for real-time and point-of-care analysis of uric acid.
![]() |
As can be seen from Table 2, μPADs are a serious competitor to other microfluidic devices for the point-of-care determination of uric acid. Obviously, they are mostly cheaper than 3D-printed microfluidic devices.[28,29] However, 3D-printed microfluidic devices have some advantages over μPADs that cannot be ignored. 3D-printing produces highly reproducible microfluidic devices, whereas it is almost impossible to create μPADs with the same physicochemical properties due to the complex nature of paper chemistry. On the other hand, the moisture content of paper material changes rapidly in different weather conditions and can seriously affect its capillary characteristics, as the basic mechanism for paper-based microfluidic analysis. Additionally, being rigid materials, they are more robust and efficient than paper-based microfluidic devices (paper is flexible and its shape changes with moisture).
Interference study
Other natural compounds present in the urine can have adverse effects on the colorimetric determination of uric acid content. To evaluate the applicability of the prosed method to real samples with complex matrix, uric acid was determined in the presence of various possible interfering compounds. The results are presented in Table 3, and show that most of the tested species did not interfere with the uric acid, even in high concentration levels (up to 110 times). However, ascorbic acid showed an interference effect at a concentration ratio of 40, likely due to its reducing potential. However, such a high concentration is unlikely to occur because the normal range of uric acid and ascorbic acid in urine is 35–72 and 6–20 mg L−1, respectively, which is far from the tested ratio of 40. However, suppose the concentration of ascorbic acid is too high (for any reason) to interfere with uric acid. In that case, it can be easily removed from the urine sample by bubbling air into the sample solution.
![]() |
Conclusion
A smartphone-based colorimetric method using a 3D-printed microfluidic device was successfully applied for the quantification of urinary uric acid. The smartphone-based color imaging and data processing provide a simple digital readout for accurate colorimetric determination without the need for sophisticated spectrophotometric detectors. The central composed design was used to optimize the influential variables to minimize the number of experiments in the optimization process, as well as to reveal the significance of the variable and their interactions. Under the optimized conditions, low LOD, wide LDR, and acceptable recovery and reproducibility were achieved. The results showed that the suggested device and strategy can be utilized as a simple, low-cost, and easy-to-operate alternative for POCT analysis of biomarkers in human urine, to be used for evaluation of renal function and monitor kidney function.
Supplementary material
Supplementary material is available online.
Data availability
The data that support this study are available in the article and accompanying online supplementary material.
Conflicts of interest
The authors declare no conflicts of interest.
Declaration of funding
The authors received no financial support for the research, authorship, and/or publication of this article.
References
[1] MI Khan, Q Zhang, YX Wang, S Saud, WW Liu, SR Liu, et al. Portable electrophoresis titration chip model for sensing of uric acid in urine and blood by moving reaction boundary. Sensor Actuators B Chem 2019, 286, 9.| Portable electrophoresis titration chip model for sensing of uric acid in urine and blood by moving reaction boundary.Crossref | GoogleScholarGoogle Scholar |
[2] N Nakanishi, M Okamoto, H Yoshida, Y Matsuo, K Suzuki, K Tatara, Serum uric acid and risk for development of hypertension and impaired fasting glucose or type ii diabetes in japanese male office workers. Eur J Epidemiol 2003, 18, 523.
| Serum uric acid and risk for development of hypertension and impaired fasting glucose or type ii diabetes in japanese male office workers.Crossref | GoogleScholarGoogle Scholar |
[3] JC Chen, HH Chung, CT Hsu, DM Tsai, AS Kumar, JM Zen, A disposable single-use electrochemical sensor for the detection of uric acid in human whole blood. Sensor Actuators B Chem 2005, 110, 364.
| A disposable single-use electrochemical sensor for the detection of uric acid in human whole blood.Crossref | GoogleScholarGoogle Scholar |
[4] NE Azmi, NI Ramli, J Abdullah, MA Abdul Hamid, H Sidek, S Abd Rahman, et al. A simple and sensitive fluorescence based biosensor for the determination of uric acid using H2O2-sensitive quantum dots/dual enzymes. Biosens Bioelectron 2015, 67, 129.
| A simple and sensitive fluorescence based biosensor for the determination of uric acid using H2O2-sensitive quantum dots/dual enzymes.Crossref | GoogleScholarGoogle Scholar |
[5] V Pavlíček, P Tůma, J Matějčková, E Samcová, Very fast electrophoretic determination of creatinine and uric acid in human urine using a combination of two capillaries with different internal diameters. Electrophoresis 2014, 35, 956.
| Very fast electrophoretic determination of creatinine and uric acid in human urine using a combination of two capillaries with different internal diameters.Crossref | GoogleScholarGoogle Scholar |
[6] D Remane, S Grunwald, H Hoeke, A Mueller, S Roeder, M von Bergen, et al. Validation of a multi-analyte HPLC-DAD method for determination of uric acid, creatinine, homovanillic acid, niacinamide, hippuric acid, indole-3-acetic acid and 2-methylhippuric acid in human urine. J Chromatogr B 2015, 998–999, 40.
| Validation of a multi-analyte HPLC-DAD method for determination of uric acid, creatinine, homovanillic acid, niacinamide, hippuric acid, indole-3-acetic acid and 2-methylhippuric acid in human urine.Crossref | GoogleScholarGoogle Scholar |
[7] D Martinez-Pérez, ML Ferrer, CR Mateo, A reagent less fluorescent sol–gel biosensor for uric acid detection in biological fluids. Anal Biochem 2003, 322, 238.
| A reagent less fluorescent sol–gel biosensor for uric acid detection in biological fluids.Crossref | GoogleScholarGoogle Scholar |
[8] D Lakshmi, MJ Whitcombe, F Davis, PS Sharma, BB Prasad, Electrochemical detection of uric acid in mixed and clinical samples: A review. Electroanalysis 2011, 23, 305.
| Electrochemical detection of uric acid in mixed and clinical samples: A review.Crossref | GoogleScholarGoogle Scholar |
[9] EFM Gabriel, PT Garcia, TMG Cardoso, FM Lopes, FT Martins, WKT Coltro, Highly sensitive colorimetric detection of glucose and uric acid in biological fluids using chitosan-modified paper microfluidic devices. Analyst 2016, 141, 4749.
| Highly sensitive colorimetric detection of glucose and uric acid in biological fluids using chitosan-modified paper microfluidic devices.Crossref | GoogleScholarGoogle Scholar |
[10] Y Yao, C Zhang, A novel screen-printed microfluidic paper-based electrochemical device for detection of glucose and uric acid in urine. Biomed Microdevices 2016, 18, 92.
| A novel screen-printed microfluidic paper-based electrochemical device for detection of glucose and uric acid in urine.Crossref | GoogleScholarGoogle Scholar |
[11] A Naccarato, A Tassone, S Moretti, R Elliani, F Sprovieri, N Pirrone, et al. A green approach for organophosphate ester determination in airborne particulate matter: Microwave-assisted extraction using hydroalcoholic mixture coupled with solid-phase microextraction gas chromatography-tandem mass spectrometry. Talanta 2018, 189, 657.
| A green approach for organophosphate ester determination in airborne particulate matter: Microwave-assisted extraction using hydroalcoholic mixture coupled with solid-phase microextraction gas chromatography-tandem mass spectrometry.Crossref | GoogleScholarGoogle Scholar |
[12] H Sohrabi, N Bolandi, A Hemmati, S Eyvazi, S Ghasemzadeh, B Baradaran, et al. State-of-the-art cancer biomarker detection by portable (bio) sensing technology: A critical review. Microchem J 2022, 177, 107248.
| State-of-the-art cancer biomarker detection by portable (bio) sensing technology: A critical review.Crossref | GoogleScholarGoogle Scholar |
[13] C-C Tseng, R-J Yang, W-J Ju, L-M Fu, Microfluidic paper-based platform for whole blood creatinine detection. Chem Eng J 2018, 348, 117.
| Microfluidic paper-based platform for whole blood creatinine detection.Crossref | GoogleScholarGoogle Scholar |
[14] L-M Fu, C-C Tseng, W-J Ju, R-J Yang, Rapid paper-based system for human serum creatinine detection. Inventions 2018, 3, 34.
| Rapid paper-based system for human serum creatinine detection.Crossref | GoogleScholarGoogle Scholar |
[15] EL Rossini, MI Milani, E Carrilho, L Pezza, HR Pezza, Simultaneous determination of renal function biomarkers in urine using a validated paper-based microfluidic analytical device. Anal Chim Acta 2018, 997, 16.
| Simultaneous determination of renal function biomarkers in urine using a validated paper-based microfluidic analytical device.Crossref | GoogleScholarGoogle Scholar |
[16] X Chen, J Chen, F Wang, X Xiang, M Luo, X Ji, et al. Determination of glucose and uric acid with bienzyme colorimetry on microfluidic paper-based analysis devices. Biosens Bioelectron 2012, 35, 363.
| Determination of glucose and uric acid with bienzyme colorimetry on microfluidic paper-based analysis devices.Crossref | GoogleScholarGoogle Scholar |
[17] S Waheed, JM Cabot, NP Macdonald, T Lewis, RM Guijt, B Paull, et al. 3D printed microfluidic devices: Enablers and barriers. Lab Chip 2016, 16, 1993.
| 3D printed microfluidic devices: Enablers and barriers.Crossref | GoogleScholarGoogle Scholar |
[18] J de Jong, RGH Lammertink, M Wessling, Membranes and microfluidics: A review. Lab Chip 2006, 6, 1125.
| Membranes and microfluidics: A review.Crossref | GoogleScholarGoogle Scholar |
[19] C Calderilla, F Maya, V Cerdà, LO Leal, 3D printed device including disk-based solid-phase extraction for the automated speciation of iron using the multisyringe flow injection analysis technique. Talanta 2017, 175, 463.
| 3D printed device including disk-based solid-phase extraction for the automated speciation of iron using the multisyringe flow injection analysis technique.Crossref | GoogleScholarGoogle Scholar |
[20] S Keshan Balavandy, F Li, NP Macdonald, F Maya, AT Townsend, K Frederick, et al. Scalable 3D printing method for the manufacture of single-material fluidic devices with integrated filter for point of collection colourimetric analysis. Anal Chim Acta 2021, 1151, 238101.
| Scalable 3D printing method for the manufacture of single-material fluidic devices with integrated filter for point of collection colourimetric analysis.Crossref | GoogleScholarGoogle Scholar |
[21] K Dalvand, SK Balavandy, F Li, M Breadmore, A Ghiasvand, Optimization of smartphone-based on-site-capable uranium analysis in water using a 3D printed microdevice. Anal Bioanal Chem 2021, 413, 3243.
| Optimization of smartphone-based on-site-capable uranium analysis in water using a 3D printed microdevice.Crossref | GoogleScholarGoogle Scholar |
[22] SH Heo, S-H Lee, High levels of serum uric acid are associated with silent brain infarction. J Neurol Sci 2010, 297, 6.
| High levels of serum uric acid are associated with silent brain infarction.Crossref | GoogleScholarGoogle Scholar |
[23] MA Bezerra, RE Santelli, EP Oliveira, LS Villar, LA Escaleira, Response surface methodology (RSM) as a tool for optimization in analytical chemistry. Talanta 2008, 76, 965.
| Response surface methodology (RSM) as a tool for optimization in analytical chemistry.Crossref | GoogleScholarGoogle Scholar |
[24] ST Narenderan, SN Meyyanathan, VVSR Karri, Experimental design in pesticide extraction methods: A review. Food Chem 2019, 289, 384.
| Experimental design in pesticide extraction methods: A review.Crossref | GoogleScholarGoogle Scholar |
[25] M Ahmadi, F Vahabzadeh, B Bonakdarpour, E Mofarrah, M Mehranian, Application of the central composite design and response surface methodology to the advanced treatment of olive oil processing wastewater using fenton’s peroxidation. J Hazard Mater 2005, 123, 187.
| Application of the central composite design and response surface methodology to the advanced treatment of olive oil processing wastewater using fenton’s peroxidation.Crossref | GoogleScholarGoogle Scholar |
[26] M Akram, MN Asghar, M Saleem Khan, S Shahid, HM Abdur Rahman, I Nadeem, Development and validation of an economical uric acid-Fe3+/Fe2+-ferrozine-based colorimetric assay to estimate uric acid level of pure and biological samples. Biosci Biotechnol Biochem 2020, 84, 1967.
| Development and validation of an economical uric acid-Fe3+/Fe2+-ferrozine-based colorimetric assay to estimate uric acid level of pure and biological samples.Crossref | GoogleScholarGoogle Scholar |
[27] G Demirel, E Babur, Vapor-phase deposition of polymers as a simple and versatile technique to generate paper-based microfluidic platforms for bioassay applications. Analyst 2014, 139, 2326.
| Vapor-phase deposition of polymers as a simple and versatile technique to generate paper-based microfluidic platforms for bioassay applications.Crossref | GoogleScholarGoogle Scholar |
[28] E Vidal, AS Lorenzetti, AG Lista, CE Domini, Micropaper-based analytical device (μPAD) for the simultaneous determination of nitrite and fluoride using a smartphone. Microchem J 2018, 143, 467.
| Micropaper-based analytical device (μPAD) for the simultaneous determination of nitrite and fluoride using a smartphone.Crossref | GoogleScholarGoogle Scholar |
[29] AS Tsagkaris, D Migliorelli, L Uttl, D Filippini, J Pulkrabova, J Hajslova, A microfluidic paper-based analytical device (μPAD) with smartphone readout for chlorpyrifos-oxon screening in human serum. Talanta 2021, 222, 121535.
| A microfluidic paper-based analytical device (μPAD) with smartphone readout for chlorpyrifos-oxon screening in human serum.Crossref | GoogleScholarGoogle Scholar |