Observations of a rotating pyroconvective plume
Neil P. Lareau A * , Craig B. Clements B , Adam Kochanski B , Taylor Aydell B , Andrew T. Hudak C , T. Ryan McCarley
A
B
C
D
E
Abstract
There is an ongoing need for improved understanding of wildfire plume dynamics.
To improve process-level understanding of wildfire plume dynamics including strong (>10 m s−1) fire-generated winds and pyrocumulus (pyroCu) development.
Ka-band Doppler radar and two Doppler lidars were used to quantify plume dynamics during a high-intensity prescribed fire and airborne laser scanning (ALS) to quantify the fuel consumption.
We document the development of a strongly rotating (>10 m s−1) pyroCu-topped plume reaching 10 km. Plume rotation develops during merging of discrete plume elements and is characterised by inflow and rotational winds an order of magnitude stronger than the ambient flow. Deep pyroCu is initiated after a sequence of plume-deepening events that push the plume top above its condensation level. The pyroCu exhibits a strong central updraft (~35 m s−1) flanked by mechanically and evaporative forced downdrafts. The downdrafts do not reach the surface and have no impact on fire behaviour. ALS data show plume development is linked to large fuel consumption (~20 kg m−2).
Interactions between discrete plume elements contributed to plume rotation and large fuel consumption led to strong updrafts triggering deep pyroCu.
These results identify conditions conducive to strong plume rotation and deep pyroCu initiation.
Keywords: field experiment, fire behaviour, fuel consumption, plume dynamics, plume rotation, pyrocumulonimbus, updraft.
Introduction
The processes driving extreme wildland fires must be understood and adequately modelled to safely fight fires and predict their societal, ecological and climate impacts. One key uncertainty in any wildland fire is the extent of feedbacks between the fire-generated winds, arising owing to the fire’s heating, and the fire spread. Fire-generated winds comprise inflows and updrafts that develop in response to the fire’s heating of the air, which results in vertical buoyant accelerations and horizontal pressure gradient forces. These fire-generated winds can drastically modify the ambient winds affecting fire spread, and even become the dominant driver of wildland fire (e.g. Coen et al. 2018), a process known as fire-atmosphere coupling. While the coupling of the fire to its own wind field is widely understood to exist, and forms the basis of coupled fire-atmosphere models (Clark et al. 1996), we still cannot reliably predict when fire-generated winds will become important in real-world fires, especially as it relates to transitions in the magnitude of the coupling and the potential for extreme fire behaviors such as destructive fire-generated tornadic vortices (FGTVs, Lareau et al. 2018, 2022), extreme updrafts supporting long range spotting (Rodriguez et al. 2020), and pyrocumulonimbus (pyroCb) capable of producing lightning, hail, and downdrafts (Fromm et al. 2006, 2010; Lareau and Clements 2016; Peterson et al. 2017a, 2017b; Lee et al. 2023).
Contributing to this predictive and knowledge deficit are insufficient observations of fire-generated winds during large and high-intensity wildland fires. This contrasts with observationally based model validation data sets available for smaller grass fires (e.g. Clements et al. 2007, 2019) and with rich observational data sets that have advanced our understanding of other high-impact atmospheric processes, such as severe thunderstorms and tornados (e.g. Rasmussen et al. 1994; Wurman et al. 2012). Although recent field campaigns are starting to chip away at this observational deficit (Coen et al. 2004; Clements et al. 2018; McCarthy et al. 2018), there are still fairly few available process-level observations offering glimpses of the magnitude and spatial extents of fire-generated winds and their impact on fire spread. One reason for this persistent observational deficit is the difficulty and hazards associated with placing research instrumentation and personnel proximal to large and active wildland fires. As a result, it is challenging to validate emerging forecasting tools that may improve predictions of high impact wildfires, including pyroCb initiation (Tory and Kepert 2021), long-range spotting behavior (Kepert et al. 2024), smoke injection height (Jones et al. 2022) and, more broadly, coupled fire-atmosphere simulatiions. It is important, for example, to have sufficient observations to evaluate not only the simulated fire spread (Shamsaei et al. 2023) but also the representation of the fire generated winds, plume dynamics, and heat fluxes from large fires (e.g. Lee et al. 2023, Roberts et al. 2024). (Shamsaei et al. 2023).
To help fill these observational and knowledge gaps, this paper provides a unique set of case-study observations that elucidate and quantify three important aspects of fire-generated circulations: (1) the development of rotation in wildfire plumes, (2) pyrocumulus (pyroCu) initiation and vertical development linked to fire-induced updrafts, and (3) the link between fuel consumption and fire-induced circulations. This is accomplished by examining remote sensor and in situ observations during a high-intensity prescribed fire that was part of the Fire and Smoke Evaluation Experiment (FASMEE; Prichard et al. 2019) in the Fishlake National Forest (FNF) of Utah, USA. Specifically, we leverage scanning Doppler lidar and radar observations that provide details of pyroCu and plume dynamics, including the development of rotation within the convective column and the structure of extreme fire-induced updrafts aloft. These data are subsequently contextualised with pre- and post-fire observations of fuel consumption within the burn plot. The results, while case-specific, nevertheless provide useful new data points as we consider the broader scope of fire–atmosphere interactions occuring during large wildland fires.
Background
To contextualise our new fire-generated wind observations, we first provide a review of previous observations of (1) plume rotation, (2) fire-induced updrafts, and (3) pyroCu and pyroCb processes.
Strong rotation, also known as a vortex, near the base of wildland and man-made convective plumes has been observed and simulated in several cases. Here, the term vortex refers to a coherent region of fluid rotation about a central axis, with an emphasis on predominantly vertical axes of rotation (though there are a range of vortex orientations possible). Vortex morphologies include whole-column rotation, counter-rotating vortex pairs and wake-like vortices (Church et al. 1980; Forthofer and Goodrick 2011; Tohidi et al. 2018). Whole-column rotation typically refers to the upright smoke- and ash-filled convective column rotating coherently in one direction. Counter-rotating vortex pairs are associated with plumes exposed to a cross wind and are often apparent embedded in the main updrafts of bifurcating plumes (Fric and Roshko 1994; Forthofer and Goodrick 2011), including plumes producing tornadic vortices (Lareau et al. 2022). Shedding vortices also occur in plumes exposed to cross winds, but these vortices detach from the fire and can translate downwind while remaining attached to the underside of the plume aloft. Vortices can both contain flaming gases (e.g. a ‘fire whirl’) or combustion by-products, such as a rotating smoke plume or column, and are important in wildland fire because they can enhance combustion rates, drive unexpected fire spread, enhance updrafts and loft embers. Excellent reviews of vortices in wildland fire are available from Forthofer and Goodrick (2011) and Tohidi et al. (2018).
Quantification of vortex strength during real-world fires requires measuring the horizontal wind component that is tangential to a contour defining the vortex perimeter. Depending on the study, vortex strength has been expressed as (1) the tangential wind difference spanning the vortex ΔVT = Vx − Vn,where Vx,n are the maximum in- and out-bound tangential winds (Marquis et al. 2008), (2) the rotational velocity Vrot = ½(Vx − Vn) (Gibbs 2016), and (3) the estimated relative vorticity ζ = 2 ΔVT/D where D is the vortex diameter (Bluestein et al. 2004). We note that ζ is sensitive to vortex diameter (i.e. for a given wind, the vorticity increases as the diameter decreases), whereas Vrot more directly represents the actual strength of the wind impacting the fire and vegetation. We also note that Vrot has gained wide use in identifying and quantifying tornados using radar observations in the severe weather community (e.g. Smith et al. 2020)
Banta et al. (1992) provide the first Doppler lidar derived quantification of whole-column plume rotation in a prescribed fire plume that induced a pyroCu cloud aloft. The rotation was fairly weak (relative vorticity ~0.02 s−1) with maximum ΔVT of 8–14 m s−1 with a plume diameter of ~750 m. Stronger but narrower (~150 m diameter) whole-column rotation reaching ζ ≅ 0.4 s−1 and ΔVT ~30 m s−1 is documented in Clements et al. (2018, see their fig. 5). In that case, lidar scans also show the vortex strength and diameter increase following the merger of smaller vortices leading to vortex growth and intensification. McCarthy et al. (2018) also report whole-column rotation during a prescribed fire observed with deployable X-band Doppler radar, finding ΔVT of ~15 m s−1 with rotation extending from the surface up to ~1200 m above ground level (AGL).
In addition to observations with deployable remote sensors, fixed-site weather radars have recently been used to document tornado-strength vortices in convective plumes from large wildfires. Lareau et al. (2018) found whole-column rotation in a pyroCb-topped plume during the Carr Fire, with radar-observed ΔVT of >70 m s−1 (i.e. rotational wind of 38 m s−1), estimated surface winds >60 m s−1 (140 miles per hour) and rotation detectable up to ~5 km AGL. In that case, the vortex spin-up coincides with rapid plume deepening due to contemporaneous pyroCb initiation. PyroCb initiation and vertical development were also observed in three cases with fire-generated tornadic vortices (FGTVs) in 2020 in North America (Lareau et al. 2022). Those cases each feature kilometre-scale counter-rotating vortex pairs associated with deep convective plumes interacting with ambient cross winds, which yields both embedded and shedding type tornado-strength vortices.
The few available observations of wildland fire updrafts indicate extreme vertical velocities extending from the surface through a deep layer (e.g. up to 5 km above mean sea level (MSL)). Videographic flow retrievals from infrared (IR) cameras reveal updrafts reaching ~60 m s−1 immediately above crown fires linked to sensible heat fluxes as large as 1 MW m−2 (Coen et al. 2004). Lidar retrievals in Banta et al. (1992) show strong updrafts extending far above the surface, reaching ~24 m s−1 near pyroCu cloud base ~2 km aloft. Even stronger updrafts, reaching ~60 m s−1 3 km AGL, are evidenced in Rodriguez et al. (2020) in association with a rapidly developing pyroCb. Although these observations provide ‘snap-shot’ evidence for the extreme nature of wildfire plumes capped with pyroCu/Cb, we lack a time-evolving perspective on plume updrafts as they vary with other fire processes, including the nature of the combustion.
Pyrocumulus (pyroCu) are a type of convective cloud that forms when heat released from wildfires produces vertically growing convective plumes that cool to their condensation temperature aloft. Pyrocumulonimbus (pyroCb), which are also a type of convective cloud possessing ice aloft, may subsequently form if the cloud reaches its level of free convection, thus releasing moist instability aloft and ascending into the upper troposphere or lower stratosphere (UTLS) where they detrain significant quantities of smoke (Peterson et al. 2018). The amount of fire heating required for plumes to grow deep enough to initiate pyroCb has recently been quantified as the ‘fire-power threshold’ based on the ambient thermodynamic profile (Tory et al. 2018; Tory and Kepert 2021). The thermodynamic environment conducive to pyroCb is like that of dry thunderstorms, often characterised by a deep well-mixed layer with large surface dew point depressions and a near-saturated layer aloft conducive to moist instability (Peterson et al. 2018). We note that pyroCu and pyroCb are both visually identified cloud types, which may be distinct from the actual fire–atmosphere coupling process of a fire-generated thunderstorm, which can include dangerous downdrafts.
The microphysics of pyroCu and pyroCb differ from ordinary clouds owing to their highly polluted nature, yielding a preponderance of small cloud droplets and ice particles that alter their satellite observed reflectance and infrared signatures (Peterson et al. 2017a). PyroCb can produce precipitation and lightning (Lang et al. 2014; Dowdy et al. 2017; LaRoche and Lang 2017) and their rapid vertical growth due to moist instability has been linked to the onset of pyrogenetic tornadoes (Fromm et al. 2006; McRae et al. 2013; Lareau et al. 2018, 2022), though it is important to note that pyroCb do not always produce extreme feedbacks on the fire environment. To this end, there remain few process-level observations of clouds growing into deep pyroCu or pyroCb and there are many open scientific questions about their structure, evolution and composition, and their role in fire–atmosphere coupling and feedback processes (Fromm et al. 2022). For example, to date, only one study provides direct observations of plume condensation levels, finding that the observed cloud base resides near the convective condensation level (CCL; Lareau and Clements 2016). The plume’s condensation level is important in that it sets the lower boundary condition for moist convection aloft but is somewhat uncertain because it results from unknown mixtures of ambient water vapour and combustion gases (Luderer et al. 2009). There are also uncertainties about the microphysics of these polluted clouds, although recent research flights provide new snapshots of pyroCu and pyroCb microphysics, showing the size and number distributions of pyrometeors below cloud base, cloud and ice concentrations in shallow pyroCu (Kingsmill et al. 2023) and ice particles in the pyroCb anvil (Peterson et al. 2022).
In the observations that follow, we add new observations and data points to each of these topics (rotation, updrafts and pyrocloud development) and compare our results with data points in the existing literature.
Material and methods
The fire, fuels and consumption
Our observations are from a FASMEE prescribed fire in the FNF of Utah, USA, occurring on 7 November 2019. The fire, referred to here as the ‘Langdon Mountain Burn’, was conducted by FNF personnel as a high-intensity stand-replacing fire designed to remove conifers to re-establish aspen groves at high elevation (~3 km MSL). The fire was ignited by a ‘helitorch’, which drops flaming fuel into the forest. Thus, the fire is not freely evolving but composed of sequential ignitions. The first ignition occurred at 11:53 Mountain Standard Time (MST) (18:53 Universal Coordinated Time (UTC)) with subsequent ignitions progressing from east to west across the unit. Ignitions continued until approximately sunset and we estimate that the period of peak burning was at ~14:30 UTC (21:30 UTC), wherein there was a large areal expanse of high-intensity fire due to the accumulation of the helitorch ignitions. There were no black-line or previously burned fuels flanking the plot. The burn unit was ~400 ha, though the total area burned on the day of the fire was not quantified in detail, and we estimate ~200 ha burned during the period of our observations.
As summarised in Ottmar et al. (2019), the burn occurred in a forest characterised by quaking aspen overstorey and subalpine fir understorey with high fuel load. Pre- and post-fire fuel surveys revealed large loading of downed woody debris (DWD) and duff on the forest floor, with estimated total fuel loads (fine, down and standing fuels) of >90 tons acre−1 (222 tons ha−1, 20.6 kg m−2). A large fraction of these fuels, up to 81 tons acre−1 (200 tons ha−1, 18.3 kg m−2), was consumed by the high-intensity fire. Within the fuel plots, the dominant DWD and duff fuel loads experienced 99 and 76% consumption respectively. These large fuel loads and fractional consumption values are evidenced in Fig. 1, which provides photographs of pre- and post-fire fuel distribution. The corresponding large heat release from the fire was the driver for the development of deep convective plumes (e.g. Fig. 2b).
Photographs pre-fire (a, c), and post-fire (b, d) from fuel survey plots on Langdon Mountain. Photo credit: Roger Ottmar.
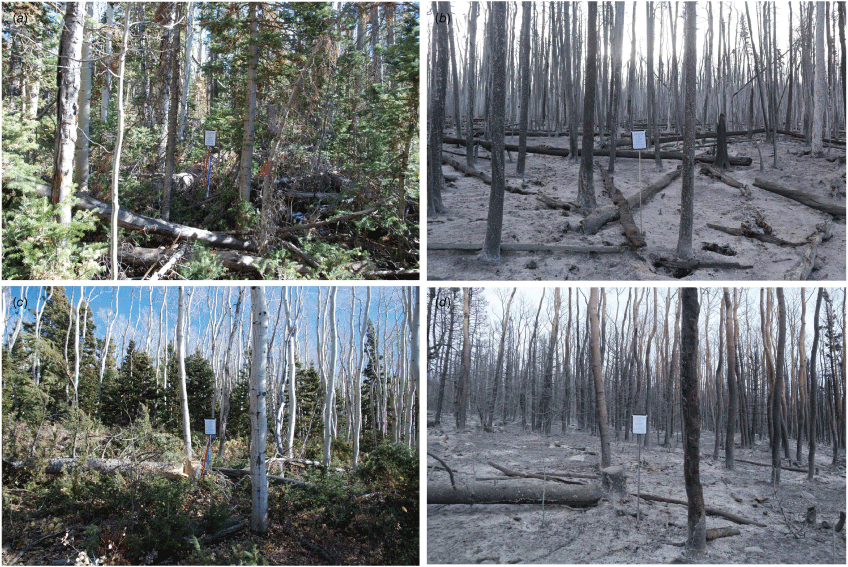
Overview of the FASMEE burn and instrumentation. (a) Topographic map of the Langdon Mountain Burn including terrain contours, approximate fire perimeter (red), and instrument scan sectors (according to the legend). (b) Photograph showing the San Jose State University (SJSU) lidar truck (left) and radar (right) with deep plume development in the background. (c) University of Nevada, Reno (UNR) lidar site. (d) Photograph of the plume near the end of the day from the UNR lidar site.
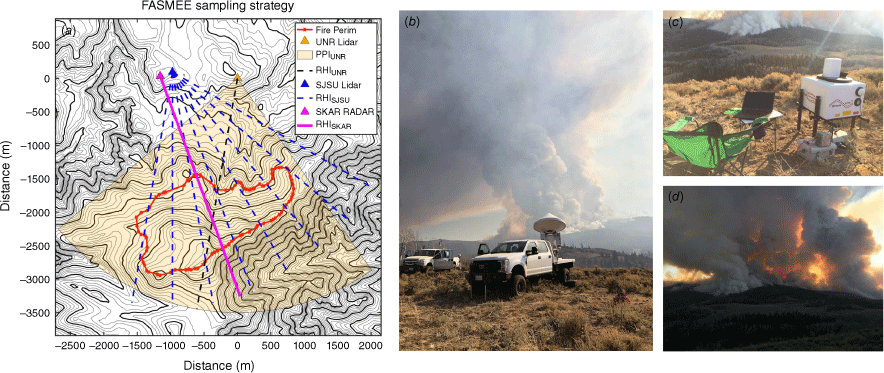
In addition to manual fuel plot surveys, pre- and post-fire airborne laser scanning (ALS) data reveal the spatial distribution of consumption and consumed percentage throughout the burn unit. Following the methods in McCarley et al. (2020, 2022), these ALS data are fitted with fuel plot observations on the ground (e.g. those in Fig. 1) to produce maps of estimated fuel loads both pre- and post-fire, which subsequently can be differenced to provide mapped consumption estimates. The ALS-based data indicate pockets of very large fuel consumption of >20 kg m−2, the distribution of which is examined in relation to plume processes in the Results section
Instrumentation
The Langdon Mountain Burn was instrumented with scanning remote sensors to observe the plume development. The instrument configuration is shown in Fig. 2 and primary instruments and observing strategies are described below.
Two scanning Halo PhotonicsΤΜ Doppler lidars were used, one from San Jose State University (SJSU), the other from the University of Nevada, Reno (UNR). These 1.5 μm infrared lasers provide time- and range-resolved attenuated backscatter coefficient (hereafter backscatter) and Doppler velocity data. The lidars are sensitive to submicrometre and micrometre-sized aerosol, including smoke, as well as larger ash and debris in the plume. Critical to some of the analyses, the lidar beam is attenuated in liquid water, meaning it does not penetrate pyro-convective clouds aloft, but will produce high backscatter at the cloud edge (e.g. Lareau and Clements 2016).
The SJSU Ka-band radar (8.4 mm wavelength) is a polarimetric scanning radar with a narrow beam width (0.31°). The radar samples the co- and cross-planar reflectivity from horizontally and vertically polarised beams. It also records the radial velocity, differential reflectivity, correlation coefficient and differential phase (see details in Aydell and Clements 2021). The radar is sensitive to suspended ash and debris within the plume, referred to as pyrometeors (McCarthy et al. 2019), but not to the submicrometre-diameter smoke aerosol (which is observed by the lidars). Pyrometeors have characteristic sizes in the millimetre to centimetre scale, though a well-developed understanding of pyrometeor size distributions is only beginning to emerge (e.g. Kingsmill et al. 2023). It is also important to note that the radar easily sees into the pyroclouds aloft, which contain a mixture of pyrometeors and hydrometeors (e.g. discussion Aydell and Clements 2021).
Observational strategy
An overview of the radar and lidar scan domains is provided in Fig. 2a. The UNR lidar conducted range-height-indicator (RHI) scans (i.e. fixed azimuth, varying elevation) centred on the upright portions of the isolated plumes during the early phase of the fire (~19:00–20:00 UTC; recall ignition was at ~18:50 UTC), then transitioned to hemispheric plan-position-indicator (PPI) scans (i.e. fixed elevation, varying azimuth) wherein a sector scan was conducted at 5° elevation increments from 0° to 55° above horizontal. The RHI scans were acquired at ~1 min intervals. The PPIs capture the broader plume development associated with continual helitorch ignitions throughout the afternoon. The lidar required ~5 min to complete a full sequence of hemispheric PPI scans. A few additional RHI scans were also conducted at ~21:00–21:10 UTC to document the plume’s condensation level. The SJSU lidar primarily conducted RHI scans centred on the upright portions of the plume throughout the fire. The SJSU Ka-band radar conducted RHI scans for most of the observing period, but also included some hemispheric RHI and PPI scans later in the burn (at ~21:30 UTC), which we use to produce plume volumes during the approximate period of peak burning (i.e. largest areal expanse of fire generating deep plumes). The radar RHI scans are much faster than the lidar scans, with acquisition rates of 10–15 s for a full scan.
Ancillary data
In addition to the deployed instruments, we used GOES17 IR and visible satellite imagery and reference radiosonde observations from Grand Junction, CO, which is ~300 km east of the burn. The radiosonde data are provided in Appendix 1.
Results
Plume evolution
The temporal plume evolution is established with a combination of radar (Fig. 3) and satellite analysis (Fig. 4).
Radar-derived time–height overview of the plume development. (a) Maximum reflectivity (shaded), plume top height (black line), and plume condensation level (green line). (b) 95% of radial velocity (shaded), condensation level (green line), and constant ascent lines (black dashed) for 10, 20, 40 m s−1 every 30 min. (c) 5% of radial velocity (shaded), condensation level (green line), and constant descent lines (black dashed) for −5 m s−1 every 30 min. Heights are listed in metres above sea level (MSL) and the ground surface is between 2700 and 3000 m near the plume base, such that the lowest radar data are just above the surface.
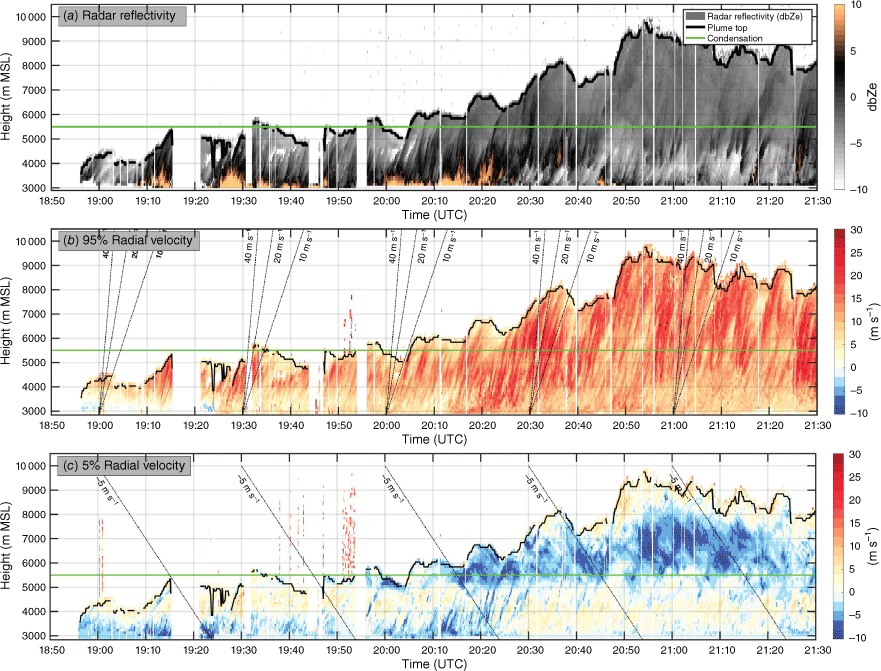
Satellite overview of the pyroCu/Cb development. (a–f) Visible satellite imagery and approximate fire contour (red line) based on a 375 K threshold at 3.9 µm. (g) Time-series of the 8.4 µm (dashed red), 3.9 µm (solid red line) brightness temperatures (left axis) along with the plume top height from the radar data (black line, right axis). The homogenous nucleation temperature (−38°C) is shown in a dashed blue line and the high t of the condensation level is shown in dashed green.
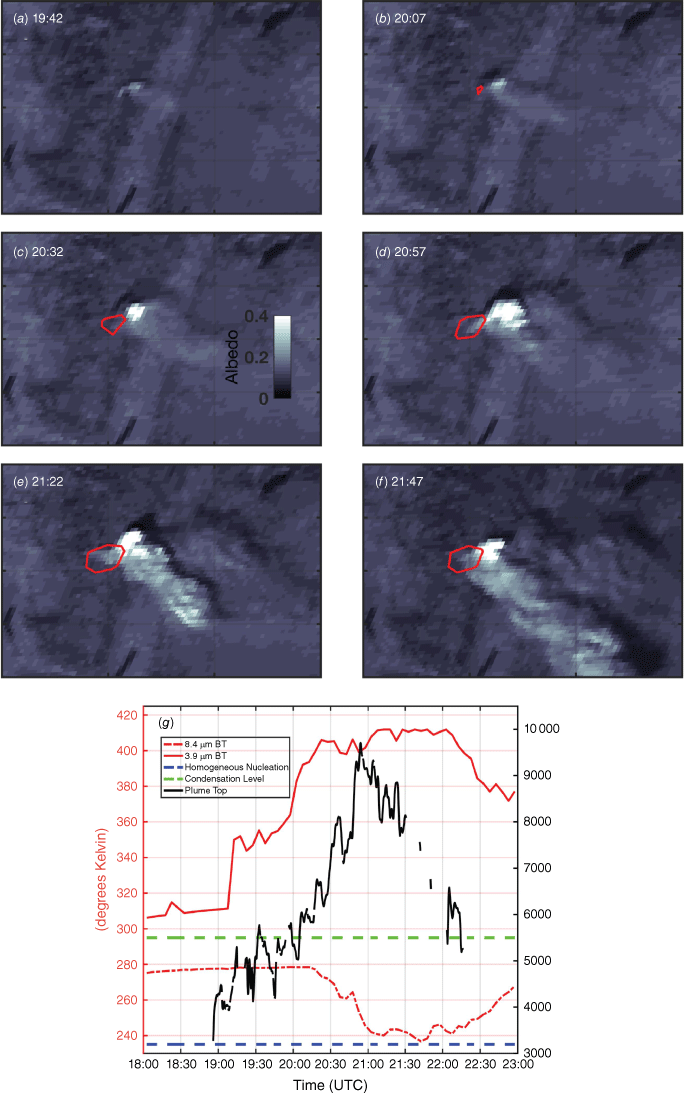
A time–height diagram of radar reflectivity (Fig. 3a, drawn from individual RHI scans) shows plume tops ascending from 4 to 5.5 km MSL (1–2.5 km AGL) in the hour following ignition (~18:50 UTC), then deepening to ~10 km MSL (7 km AGL) by 20:55 UTC via a series of step-like pulses. The condensation level, detailed later, is at ~5.5 km MSL (2.5 km AGL) such that there is considerable deep pyroCu and potentially pyroCb development by 20:20 and 20:50 UTC, respectively. These data also indicate episodic high-reflectivity cores rising through the plume, manifest as streaks sloping up and to the right due to parcel ascent with time. These reflectivity cores/streaks are likely due to higher pyrometeor loading in the more buoyant updrafts, though measurements of buoyancy are not available to test this assumption. The reduction in reflectivity in the lower plume after 20:30 UTC is in part due to the sloping nature of the convection such that the base of the plume core was not always centred in the RHI scan plane (though it is captured in volumetric scans shown later).
Fig. 3b, c shows the corresponding 95 and 5% radar radial velocities. The 95th percentile data capture the strongest outbound flows, which are dominated by updrafts. The 5th percentile data correspond to weakest outbound, or strongest inbound, flows including downdrafts. As these data are extracted from the RHI scans, the velocities at a given height are measuring different components of the flow: higher-elevation angles more closely measure the vertical velocity, whereas lower scan angles better sample horizontal flow.
The 95% velocity data indicate enhanced outbound flow (darker reds in Fig. 3b) in streaks like those in the reflectivity data, indicative of stronger updrafts in individual convective elements. The streak’s slopes (i.e. time vs height) correspond to the rise rate of the convective elements. For reference, we have added 10, 20 and 40 m s−1 constant ascent lines (black dashed lines) at 30-min intervals throughout the diagram. Most slopes are between 15 and 30 m s−1, with lower velocities close to the surface and near the plume top, due to accelerations and decelerations, respectively. The quasi-constant slopes in the mid-plume suggest an approximate balance between accelerations and decelerations in that region (e.g. buoyant acceleration vs drag forces).
The ‘downdraft’ data (i.e. 5%, Fig. 3c) also show streaky distributions and substantial variation with height. The same ‘up-and-to-the-right’ streaks are apparent in the lower plume (<5500 m), especially at 20:00–20:30 UTC. The ascent of the negative velocity features is due to mechanically forced circulations linked to the updrafts themselves. Inspection of individual RHIs (see Supplementary Video S1) shows that features are horizontal-axis vortices that are well known features of convective elements in both cumuli and wildfire plumes (Lareau and Clements 2017; Clements et al. 2018; Lareau et al. 2018; Rodriguez et al. 2020).
Above cloud base (5.5 km), broader ‘down-and-to-the-right’ swaths are observed (Fig. 3c). This diagram includes descent lines for −5 m s−1 for reference. These downdrafts within the pyroCu layer are likely linked to two sources of negative buoyancy: (1) latent cooling due to evaporation of cloud condensate, and (2) overshooting convective elements that become negatively buoyant in their stable surrounding. Notably, none of these downdrafts sink back to the surface and thus they likely do not influence fire processes. This contrasts with the idea of ‘plume collapse’ wherein strongly evaporatively cooled parcels in pyroCb with precipitation or virga can sink to ground level. Thus, even though portions of the pyroCu are subsiding and evaporating, in this case it is not ‘collapsing’. This distinction has important practical implications for firefighters who may mistake a subsiding pyroCu with a more dangerous pyroCb producing downdrafts that can impact the fire ground (e.g. Thurston et al. 2015).
GOES17 visible and IR (3.9 and 8.4 μm) imagery (Fig. 4a–f) and time series data (Fig. 4g) capture the onset and deepening of the pyroCu and the intensification of the fire corresponding to the plume deepening observed by radar. The visible imagery indicates a transition from a shallow smoke plume with shallow pyroCu (Fig. 4a, b) to growing pyroCu ~20:30 UTC (Fig. 4c). The growing pyroCu is consistent with what the World Meteorological Organisation (WMO) would categorise as a ‘cumulus congestus flammagenitus’ (WMO 2023), which some researchers and meteorologists refer to as towering or deep pyroCu. This timing corresponds with the sustained plume deepening above the condensation level apparent in the radar time–height diagram (Fig. 3a). The vertical and horizontal extent of the deepening pyroCu increases considerably at 20:57 UTC (Fig. 4d), corresponding to the period of radar-observed plume tops of ~10 km. Thereafter, a deep pyroCu (perhaps a pyroCb as discussed below) persists, with smoke, ash and cloud detraining aloft into the ambient northwesterly flow (Fig. 4e, f). We note that the lower reflectivity values during this time may be a result of RHI scans missing some of stronger convective cores near the surface, which were spread over a broader domain later in the fire, making instrument pointing more difficult.
Although we have direct measurement of the plume depth via radar, it is still useful to examine the plume top temperature via the 8.4 µm brightness temperature (blue line in Fig. 4g). The cloud top cooling starts at ~20:20 UTC, reaching a minimum temperature of 236.7 K (−36.4°C) at ~21:40 UTC, which is close to the homogeneous freezing temperature (−38°C, 235.15 K), which is the widely accepted pyroCb threshold due to de facto glaciation (Peterson et al. 2017a). It is worth noting that these GOES data likely overestimate the cloud top temperature (i.e. underestimate height) owing to the small scale of the plume relative to the large IR pixels (~2 × 2 km). Indeed, using the radar-observed plume height (~10 km) and the nearest available sounding data (Grand Junction, CO, which is ~300 km to the east), the plume may have reached −50°C (see sounding data in Fig. A1).
Such a cold cloud top associated with a spreading anvil would categorise this cloud as a pyroCb in some frameworks, for example the approach of Peterson et al. (2017a, 2017b) based on the homogeneous freezing temperature. However, there was no lightning detected or thunder heard and both radar and visual inspection did not indicate any precipitation processes, which is relevant to the preceding discussion of the absence of downdrafts reaching the surface. As such, it might be best to consider this event as culminating in towering or deep pyroCu. Accordingly, we refer to the cloud as towering pyroCu throughout the remainder of the manuscript, though reserve the possibility that this cloud may be classified as a pyroCb in some settings.
To summarise changes in the fire intensity during the plume and cloud growth, we examine the 3.9 µm brightness temperature (solid red line, Fig. 4g), which is sensitive to fire thermal emissions (Dozier 1981). These data first indicate warming beyond background temperature at 19:10 UTC, which is ~20 min after the helicopter ignition. This lag is due to the pixel resolution and sensor sensitivity, which often require the fire to grow upscale or intensify before detection, though hot fires can sometimes be detected very quickly (Lindley et al. 2016, 2020). A subsequent rapid increase in 3.9 µm brightness temperature occurs at 20:00 UTC, shortly preceding the development of the deep pyroCu. The 3.9 µm brightness temperature eventually plateaus owing to sensor saturation at ~415 K, with some decreases due to pyroCu obscuration.
Based on these combined radar and satellite data, we next subdivide our detailed plume analyses into three phases: (1) early plume rise (18:50–20:00 UTC); (2) onset of rotation (20:00–20:20 UTC); and (3) towering pyroCu processes (20:50 UTC onward).
Early plume rise
Following ignition, an isolated, single-core, upright plume developed as observed by the radar (Fig. 5a–e, k–o) and lidar (Fig. 5f–j, p–t). Note that these sensor’s RHI scan planes intersect the plume at somewhat different angles (Fig. 5u). A few key points emerge from inspection of these data.
Summary of the early plume development as observed by the SJSU radar and UNR lidar. (a–e) Radar reflectivity. (f–j) UNR lidar attenuated backscatter coefficient. (k–o) Radar radial velocity. (p–t) Lidar radial velocity. (u) Terrain map showing instrument RHI scan planes. (v) Photograph of the plume development from the UNR lidar vantage point.
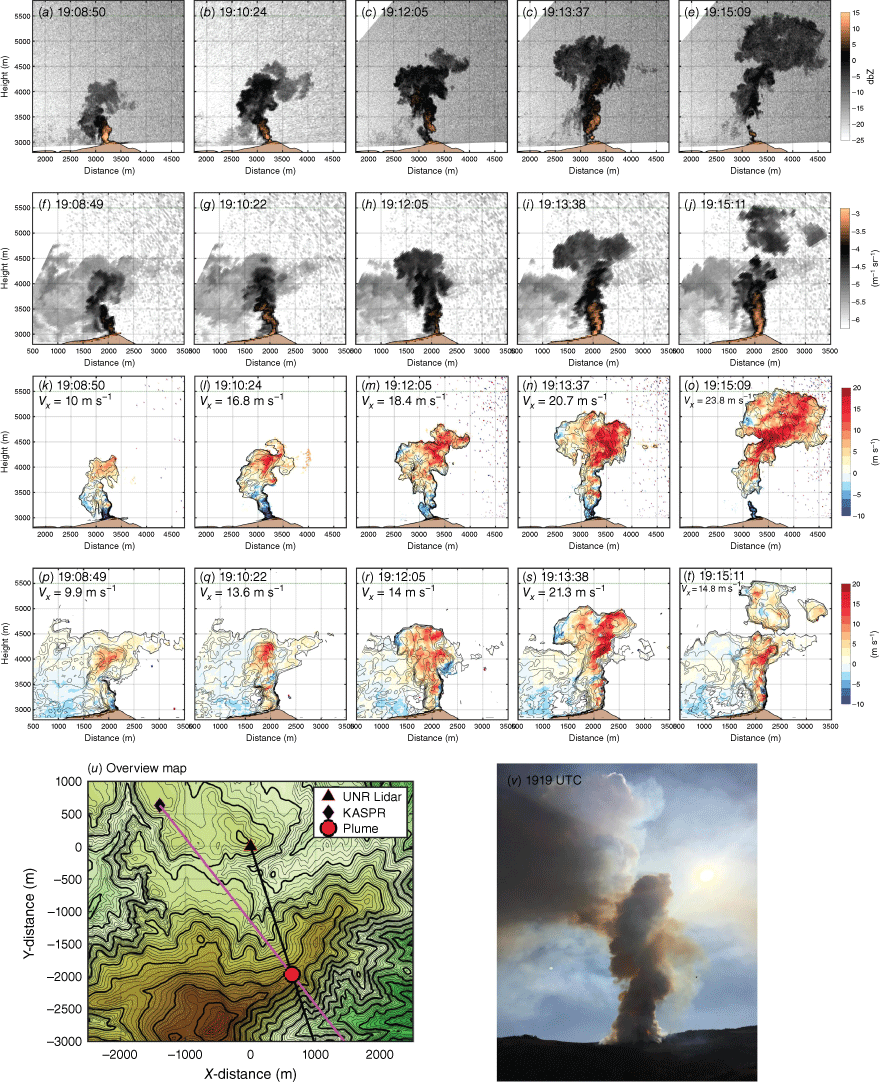
First, the radar and lidar provide similar information about the plume. Contemporaneous radar reflectivity (Fig. 5a–e) and lidar backscatter (Fig. 5f–j) both show a ‘starting plume’ (Turner 1962) characterised by: (1) a broadening ‘plume cap’ ascending gradually with time, (2) plume width broadening with height, (3) lobes of smoke and ash hanging from the plume edges (e.g. ring vortices), and (4) ash and smoke concentration decaying with height owing to entrainment dilution. Despite the different scan angles, many of these features are common between the radar and lidar, indicating a quasi-symmetric structure, which makes sense given the isolated nature of the combustion near the time of ignition.
There are, however, some differences in the radar and lidar observations including: (1) lidar sensitivity to diffuse smoke outside the plume core, and (2) differences in the upper plume due to wind shear advecting the upper plume eastward out of the lidar scan plane while remaining in the radar’s view (compare Fig. 5e, j). This shear is also evident in the photograph (Fig. 5v), where a separation between the upper and lower plume is evident. The photograph also shows a shallow (tens of metres) initial pyroCu developing at the plume top. The radar and lidar data place the plume top at ~5500 m, which we will later confirm is the plume’s condensation level.
Both sensors also reveal similar kinematic structures throughout the plume (Fig. 5k–t). For example, comparing panels n and s in Fig. 5, outbound flow >20 m s−1 along the ‘back edge’ of the plume and extending into the broadening plume cap is apparent in both the radar and lidar data. Despite these strong updrafts, the plume cap is only ascending at a rate of ~6 m s−1 (inferred from change in plume top heights between panels m and n). This is consistent with the established dynamics of a starting plume wherein ‘the cap moves with a constant fraction of the plume velocity’ (Turner 1962). To maintain mass continuity, the plume fluid must thus spread laterally at the cap, forming the broadening plume top apparent in the observations.
One final feature of note is the weak ‘clear air’ inbound flows (1–3 m s−1) apparent in the lidar observations between the lidar location and the plume base (blue shading in Fig. 5p–t). These data show that the ambient flow in this region is weak and has not yet been perturbed by the plume-base convergence, which would necessitate outbound flows (rather than the noted inbound flow) toward the plume. As we will show in the next section, as the plume further develops, a profound modification of this flow occurs.
Development of plume rotation
Amongst the notable aspects of the Langdon Mountain Burn was the development of strong ‘whole-column’ anticyclonic plume rotation and ~10-fold increase in horizontal winds near the plume base as compared with that in the ambient environment. Fig. 6 summarises the plume structure and rotation at 20:14–20:15 UTC, and a time-lapse video is provided in the Supplementary material (see Supplementary Video S1, courtesy of Holt Hanley, SJSU).
Overview of plume rotation. (a) Annotated photograph showing the anticyclonic rotation. (b) Lidar attenuated backscatter isosurfaces showing the plume structure. (c) Out-bound (red) and in-bound (blue) radial velocity isosurfaces on top of the backscatter isosurfaces. (d, e, f) Radial velocity (shaded) and backscatter (contoured) lidar PPI scans through the plume base at 5°, 10° and 15° elevation tilts.
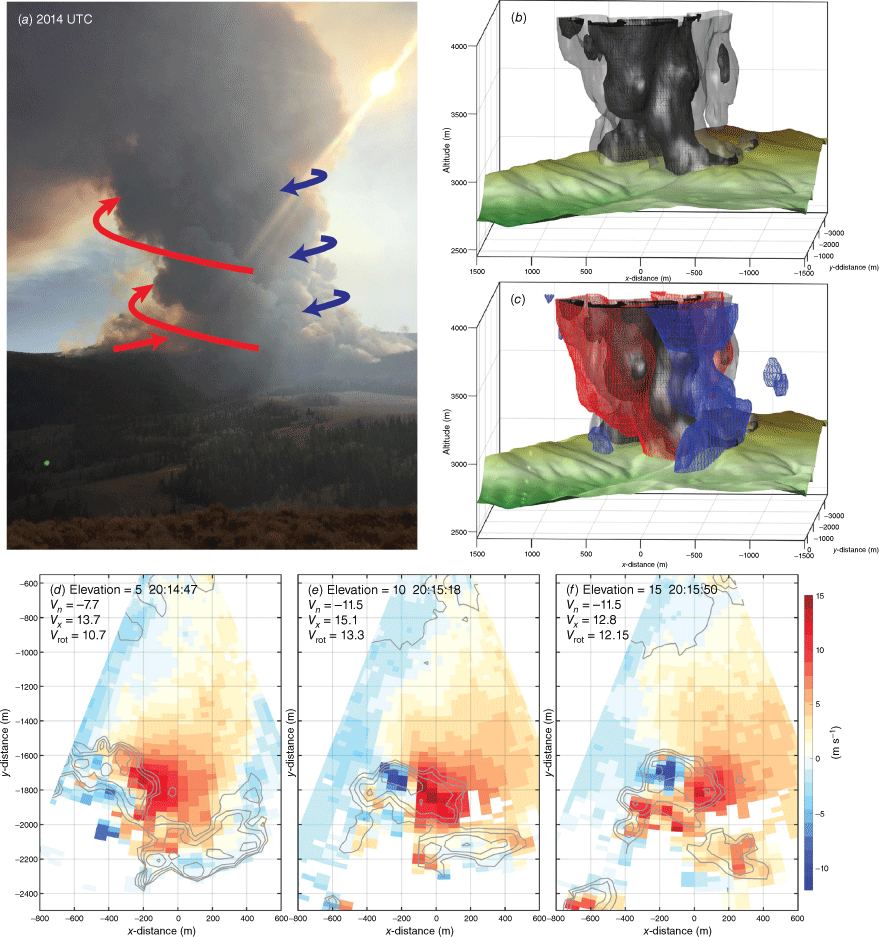
The first panel (Fig. 6a) shows a still photograph of the plume annotated with the sense of rotation derived from the video. Also apparent is the helical nature of the updraft, with individual convective components spiralling upward. The corresponding lidar-volume rendering (Fig. 6b) shows an upright plume core with a slight tilt toward viewers’ left (to the east in physical space) and two plume components: (1) the main updraft column, which is closer to the lidar, and (2) a more distant plume element wrapping around the back of the primary plume core. These features are also apparent in the photograph. The sense of rotation is subsequently visualised by including red and blue isosurfaces at 5 and −1.5 m s−1 respectively (Fig. 6c). The blue (inbound) and red (outbound) surfaces ‘hug’ the viewer’s right and left sides of the plume, respectively, indicating that the whole column is involved in distinct anticyclonic rotation centred on the plume core. The region of outbound flows is broader than the inbound flow, though we only have data where sufficient smoke and ash scatters exist, so it is possible that the inbound flow extends beyond what is shown.
Quantification of the rotation is obtained using lidar PPI snapshots at 5°, 10° and 15° elevation slices through the plume base (Fig. 6d–f). Each slice indicates a couplet of in- and out-bound motions centred on the plume core. In each panel, we also show the maximum in- and out-bound tangential velocities (Vx, Vn) and the rotational wind magnitude (Vrot, Gibbs 2016, see details in the background sections above). The strongest Vrot and Vx,n occur at the 10° elevation tilt, reaching magnitudes of 13.3 and 15.1 m s−1. These values are notable in that they are an order of magnitude stronger than the ambient winds (~1.5 m s−1 measured by the scanning lidar away from the fire and shown in Fig. 5p–t), and thus represent a significant fire-generated wind that may feed back on the fire development and intensity, though we lack observations of the combustion processes to test this hypothesis. The plume diameter is ~500 m and the tangential wind maxima are separated by ~150 m. The implied vorticity (ζ) is −0.34 s−1, which is slightly less than the 0.4 s−1 vortex documented in Clements et al. (2018) and much less than the large rotational velocities in FGTVs (e.g. Vrot 38 m s−1 in the Carr Fire; Lareau et al. 2018).
The link between the rotation and the broader plume structure is established using a combination of lidar and radar observations (Fig. 7). The time-averaged radar reflectivity and radial velocity RHIs (Fig. 7a, b) indicate the merging and contraction of two high-reflectivity plume cores in the lowest 500 m AGL. The radial velocity data confirm the converging character of the lower plume, showing ‘blue’ inbound flow in the more distant plume core and ‘red’ outbound flow in the closer plume core (Fig. 7b). Because of this merging, the plume geometry exhibits narrowing with height, reaching a ‘waist’ or ‘neck’ at ~3500 m MSL, before broadening with height aloft. We note this ‘neck’ height can be an important consideration for plume rise modelling (e.g. Tory 2018). A photograph of this merging and contracting, sometimes called ‘indrafting’ (Finney and McAllister 2011), is provided in Fig. 8 albeit at a slightly earlier time.
Summary of plume structure leading to increasing rotation. (a, b) Time-averaged radar reflectivity and radial velocity. The inset boxes in (b) are used for the in- and out-bound flow components. (c) in- and out-bound flow in the lower plume along with the estimated along-radial convergence (purple). (d–u) Lidar PPI scans for the 5° (red frames), 10° (blue frames), 15° (purple frames) lidar elevation scans. Shading shows the radial velocity and contours the attenuated backscatter coefficient. The black dashed line indicates the radar scan plane. The lidar tilts are also shown in (a) relative to the deeper plume structure.
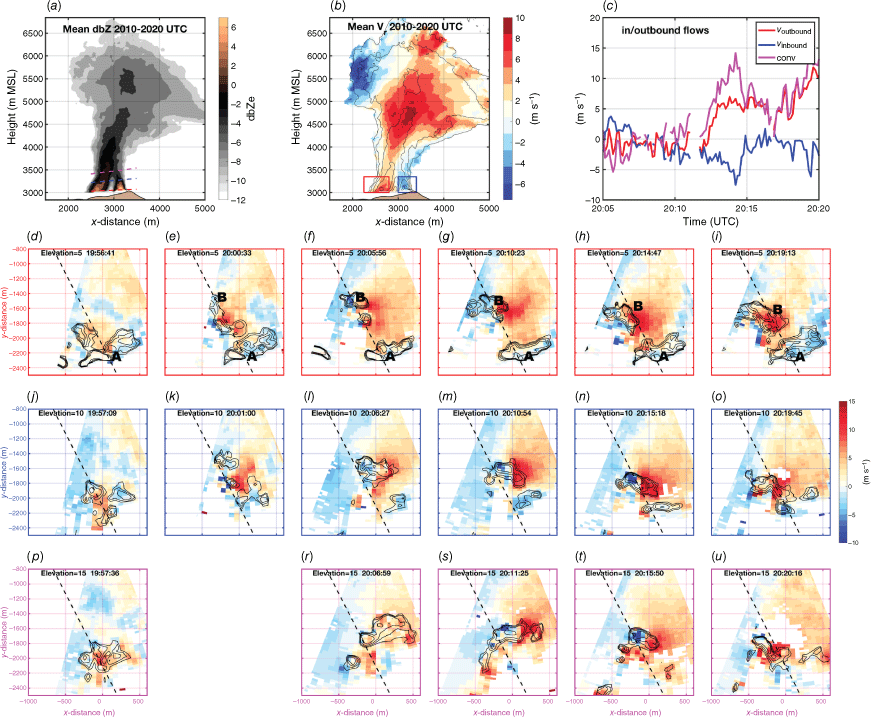
The temporal variation in the magnitude of the convergence is estimated using the mean radial velocity in two boxes centred on the merging plume elements (red and blue boxes in Fig. 7b; data shown in Fig. 7c). These velocity data show that both the outbound (red) and inbound (blue) flow components increase in magnitude between 20:11 and 20:14 UTC, reaching contemporaneous peaks of ±7 m s−1, respectively. The confluent component of the flow is approximated as the bulk difference between the in- and outbound flows (magenta line, Fig. 7c), and thus reaches a peak of ~14 m s−1.
Given the context of converging plume components, it is useful to examine the sequence of lidar radial velocity and backscatter PPI scans at successive elevation tilts (Fig. 7d–u). For ease of interpretation, the 5°, 10° and 15° elevation tilts are organised in rows, with the axes colour-coded (red, 5°; blue, 10°; magenta, 15°). There are three primary features in these data: first (moving from left to right in the figure), the strength of the rotation increases during the period of plume convergence, reaching a peak at 20:14 UTC. Second, the lidar backscatter indicates the rotation coincides with a period of interaction between plume elements (labelled A, B). Element A is a plume from the earlier ignitions whereas Element B is from a new helitorch ignition at ~20:00 UTC. This ignition rapidly intensifies, as apparent in development of higher attenuated backscatter and contemporaneous perturbation of the winds (e.g. Fig. 7e–h). As the new plume (B) develops, the older plume (A) is drawn inwards toward the new plume. This is apparent as the decreasing gap in backscatter between the plume elements with time. It is not immediately clear why ignition B increases more rapidly than previous ignitions.
The third aspect of the plume development and rotation apparent in these data is the contraction of the plume with height into the aforementioned ‘waist’ or ‘neck’ region and the associated tightening of the circulation. Taken together, these lidar PPIs show the complex fire geometry near the surface (i.e. spatial distribution of backscatter) is reduced with height, yielding a single plume core aloft with a radius of ~500 m (compare Fig. 7 panels h, n, and t). For reference, the 5°, 10° and 15° lidar scan planes are superimposed on the radar figure (Fig. 7a), showing that these features match up nicely with the contracting and merging plume elements (note that the lidar backscatter contours in Fig. 7d–i provide a proxy for the location of the active fire at this time).
Collectively, these data suggest that the interaction of plumes from discrete combustion regions (and thus complex fire line geometry) and ‘indrafting’ are important in generating the whole-column plume rotation in this case. This appears to contrast with cases of flow–plume interactions during stronger winds that seem to favour counter-rotating vortex pairs (CVPs) associated with recent FGTVs (Lareau et al. 2022). However, we lack detailed environmental wind observations that could further support or refute this hypothesis. Obtaining more comprehensive wind speed measured in complex terrain should be included in future campaigns if possible.
Towering pyroCu processes
Plume condensation level (PCL) is an important threshold and lower boundary condition for pyroCu and pyroCb initiation. We estimate the PCL by comparing lidar (Fig. 9a) and radar (Fig. 9b) volume renderings of the deep pyroCu-topped plume at ~21:30 UTC. The radar data reveal the full plume structure, including the sloping pyrometeor-laden updraft cores rising from the surface and the broadening pyroCu aloft. The lidar, in contrast, captures only the lower portions of the plume because it is attenuated in liquid water droplets above the PCL. Fig. 9c reinforces this interpretation by displaying radar and lidar maximum reflectivity (shaded) and backscatter (contoured) plume cross sections. These data indicate very good agreement in the lower plume but a contrasting representation aloft due to attenuation of the lidar beam along the cloud base and edges (e.g. ~5500 m for the downwind portion of the plume).
Summary of lidar (a), and radar (b) volumetric plume observations. (c) Maximum reflectivity (shaded) and backscatter (contoured) in the x-direction. Black dashed line shows the plume’s condensation level.
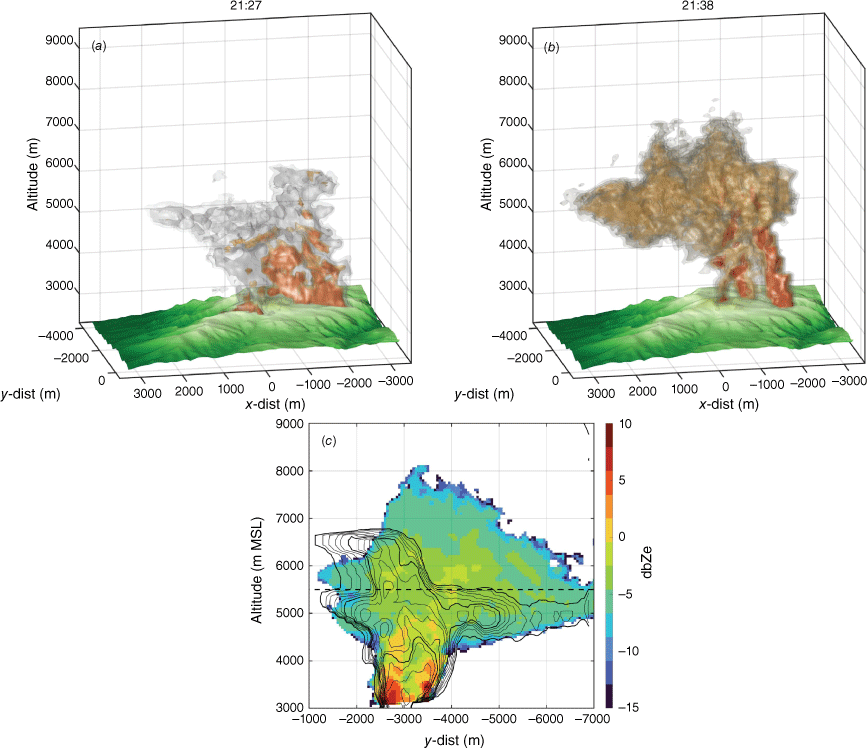
A more detailed examination of the condensation level is accomplished by extracting radar and lidar RHI scans at 21:15 UTC (Fig. 10). All three instruments show similar updraft core structure in the lower plume, but both lidars attenuate at ~5500 m in the downwind portion of the plume (green dashed line). There is a local enhancement in the lidar backscatter where it reflects from cloud droplets and before it is attenuated (see inset in Fig. 10a). This signal is similar to that observed at the PCL in other pyroCu/Cb-topped plumes (see Lareau and Clements 2016). The radar data, in contrast, span the transition into the cloud and continue to cloud top (Fig. 10b). This is because the radar beam is not attenuated in the cloud and retains strong backscatter from the mixture of pyrometeors and hydrometeors aloft.
RHI scans examining plume condensation level. (a) UNR lidar RHI scan; (b) SJSU ka-band radar RHI; (c) SJSU lidar RHI. Shown in each panel is the estimated condensation level (green dashed line). The inset panel shows the maximum UNR lidar backscatter as a function of height.
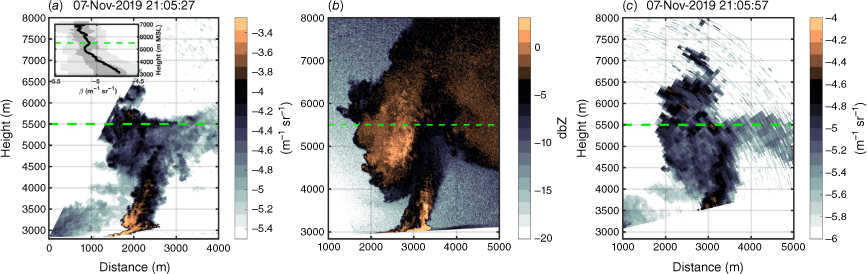
One might expect that the polarimetric radar observations would reveal changes in the shape of scatters proximal to the condensation level owing to the presence of quasi-spherical cloud droplets commingled with irregular ash particles. This is not, however, observed. As was shown in a previous analysis of polarimetric properties of the same plume in Aydell and Clements (2021), no significant change in polarimetric variables occurs above the condensation level. This lack of differentiation is attributable to the small size of the cloud droplets, and the lack of large precipitation-sized hydrometeors, as compared with the abundant larger pyrometeors lofted into the cloud by the powerful fire-generated updrafts. Thus, in the present, non-precipitating towering pyroCu, the lidar signal attenuation remains the most useful method for diagnosing cloud base. The lack of precipitation processes is also likely linked to the absence of lightning in this pyro-cloud.
The kinematics of the cloud layer are examined using the time-maximum (red/grey shades) and -minimum (blue contours) radial velocity for RHI scans spanning 20:51–21:15 UTC (Fig. 11a). These data show a kilometre-wide updraft core with outbound speeds >25 m s−1 flanked by downdrafts on the plume edges. The cellular distribution of the strongest updrafts, and assessment of corresponding animations (see Supplementary Video S2), suggests the plume possesses a ‘chain of thermals’ comprising individual buoyant elements such as occur in cumulus convection (Morrison et al. 2020). This central-updraft, flanking-downdraft structure is further reinforced by examining 3D updraft and downdraft isosurfaces (Fig. 11b) and is broadly consistent with other observations of deep wildfire convective plumes (e.g. Lareau and Clements 2017; Rodriguez et al. 2020). The maximum in-cloud outbound speed is ~35.5 m s−1 at ~6200 m MSL, indicating extreme rising motion within the cloud layer. The updrafts decay above ~7000 m MSL, likely owing to overshooting their level of neutral buoyancy and interaction with stronger winds aloft. This decay was also evident in the time–height diagram (Fig. 3b).
Overview of plume updraft and downdraft structures. (a) Time-maximum (red and grey shading) and -minimum (blue contours) radial velocity. Also shown are the reflectivity contours (black lines), the approximate updraft trajectory (white dashed line), plume condensation level (green dashed line), and radar scan radials (light grey lines). The inset axes show the maximum velocity as a function of height (red line) and the estimated flow along the plume trajectory (purple line). (b) Volume rendering of the updraft (orange and red) and downdraft (blue) isosurfaces along with the −20 dbZ reflectivity surface (transparent grey). Also shown is the estimated fuel consumption as coloured shading along the terrain surface according to the colour bar.
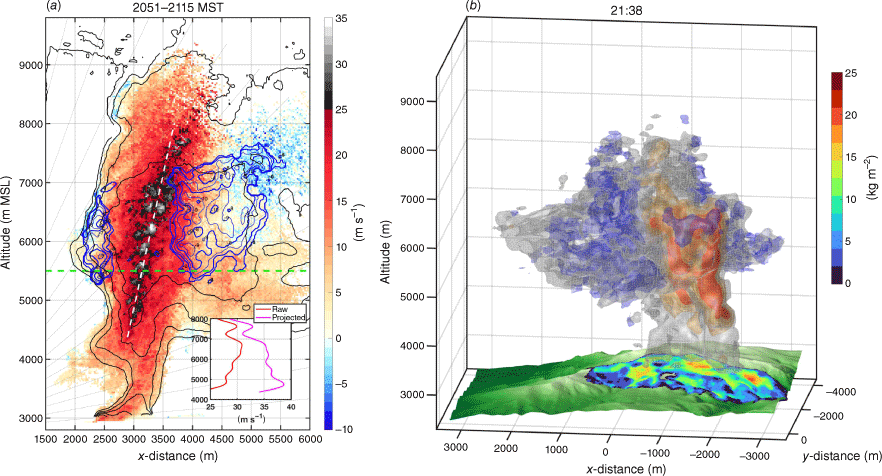
The outbound flow at cloud base is >25 m s−1, which implies considerably stronger updrafts due to the beam angle of <45° (see grey dashed lines showing beam scan angles). To account for this underestimation of the pure updraft, we use the slope of the updraft core (white dashed line Fig. 11a) and compute the flow along that line that would result in the observed radial velocity component at each height in the updraft core (inset panel). The results suggest that the sub-cloud updrafts exceed 35 m s−1. These values are contextualised by previous observations by Banta et al. (1992) and Rodriguez et al. (2020) of sub-cloud updrafts in pyroCu/Cb plumes of 24 and 30–60 m s−1, respectively. These cloud base updrafts are notable in that their inertia can overcome appreciable convective inhibition and thus drive pyroCu/Cb development even in unfavourable thermodynamic conditions.
Downdrafts (blue contours) are preferentially observed above the condensation level and along the flanks of the updraft core, with peak values of ~10–11 m s−1, which is approximately one-third of the maximum updrafts. The downdrafts along the leading plume edge are confined to a narrow (~500 m) corridor whereas the downdrafts on the downwind side of the plume occur throughout a broader ~2 km region. The occurrence of downdrafts in the cloud layer suggests a few processes likely contribute to their occurrence. First, mechanically forced horizontal axis eddies along the plume edge likely produce some of these downdrafts (inspection of Supplementary Video S1 shows these clearly). Second, evaporation into the dry air aloft (see sounding in Fig. A1) of the characteristically small pyroCu/Cb cloud droplets likely produces negative buoyancy, like subsiding shells in ordinary cumulus (e.g. Heus and Jonker 2008). Lastly, the plume is likely overshooting its level of neutral buoyancy, causing some downwind sinking of negatively buoyant plume.
Linking plume processes to fuel consumption
Fig. 12 summarises the pre-fire fuel distribution (satellite image and fuel map, Fig. 12a, b) and the spatial distribution of fuel consumption estimated from ALS observations (Fig. 12c). These data show a mix of heavier fuels (e.g. greens correspond to conifer stands intermixed with aspen overstorey in Fig. 12a) and fine fuels in meadows (beige regions in Fig. 12a). Within the forested regions, pre-fire ALS data indicate total fuel loads up to and exceeding 40 kg m−2 (colour shading Fig. 12b). The fuel consumption (Fig. 12c) indicates spatially heterogeneous consumption patterns corresponding to foci of the helitorch ignitions progressing from east to west across the burn unit. Unfortunately, we do not have quantification of the ignition timing and extent, something that future experiments should endeavour to provide. The statistical distribution of consumption is shown in the histogram in Fig. 12e. The median and mean consumption values amongst burned pixels (i.e. with non-negligible consumption) were 5.8 and 6.8 kg m−2, respectively, and more intensely burned pixels (e.g. 75% values) exhibit consumption >11 kg m−2. Notably, there are three regions (coloured outlines in Fig. 12b) with local consumption maxima >20 kg m−2.
Summary of fuel consumption and plume processes. (a) Google imagery of pre-fire fuels. (b) Terrain map overlaid with ALS-estimated pre-fire fuel loads (coloured contours). (c) ALS-estimated fuel consumption and estimated sensible heat flux (see values in parentheses). (d) Time-maximum lidar attenuated backscatter. Each panel also shows the terrain contours at 10 m (light black) and 50 m (solid black) intervals. (e) Distribution of fuel consumption and estimated fluxes.
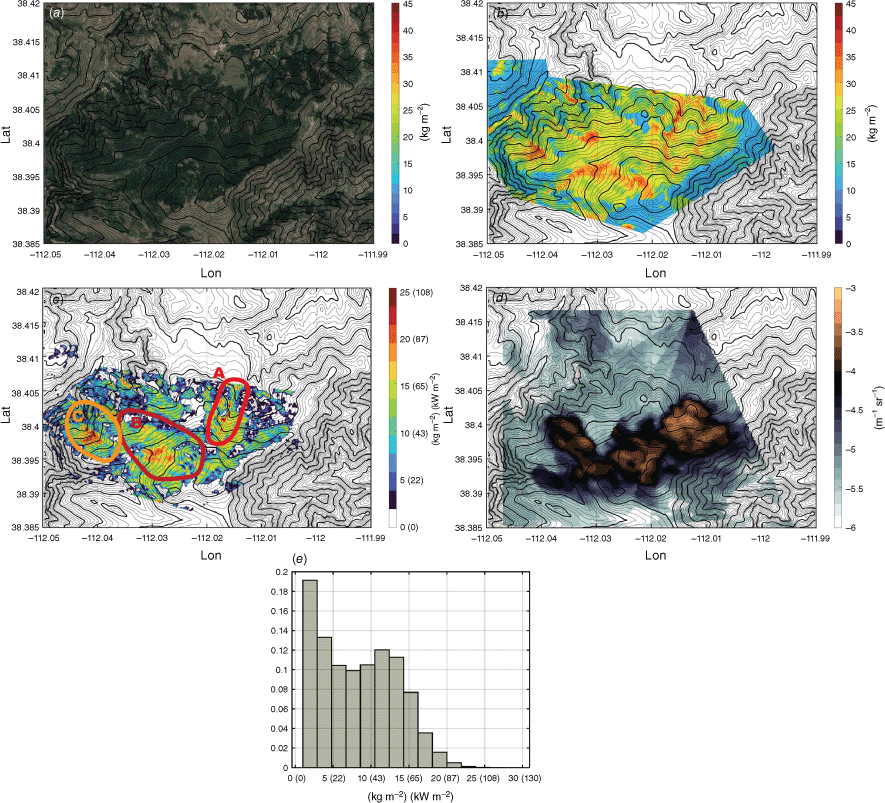
To link consumption to plume processes, we estimate the sensible heat fluxes, which were not explicitly measured. This is accomplished using the ALS-estimated fuel consumption along with an estimated consumption time scale (Δt) of ~1 h using:
where Hs is the sensible heat flux (W m−2), w is the total ALS-derived fuel consumption (kg m−2) burned in the increment Δt (3600 s), FMC is the fuel moisture content (~0.2; see fig. 18 in Ottmar et al. 2019), and h is the heat content of dry fuel (18 700 J kg−1). The sensible heat flux values, shown in parentheses for the map and the inset histogram, indicate peak fluxes of ~90 kW m−2 associated with the local maxima in consumption. These values are consistent with those estimated for other deep convective columns from wildfires (Lareau and Clements 2017). Noting that our time scale is a crude estimate, it is likely that instantaneous fluxes were much higher.
Next, we examine the spatial distribution of time-maximum lidar attenuated backscatter from the 10° elevation PPI scans throughout the burn, which are slightly above the surface (Fig. 12c). These data indicate that the local maxima in backscatter from plume cores agree well with the consumption maps, albeit with plume cores displaced somewhat east–southeast (ESE) from the consumption maxima. This ESE tilt to the plume is particularly evident in the large consumption regions in Region A, which corresponds with the region of strong rotation and the slightly tilted plume shown in Fig. 12a, b. We note that the consumption maxima in Region C are not linked to the strong backscatter signal because they occurred after the lidar and radar observations concluded for the day.
Finally, the consumption maxima in Regin B can also be directly related to vigorous plume cores observed with the radar and the deepest plumes of the afternoon. Returning to Fig. 11b, note that the updraft core locations are well correlated with the independently derived ALS consumption data, which are mapped onto the terrain using coloured markers. Note the consumption values within this region are not only large, but are also spatially expansive compared with the values in Region A. We hypothesise that this broad region of intense combustion was a critical factor in producing the deep pyroCu plume tops reaching 10 km and updrafts exceeding 35 m s−1. In future observing campaigns, resolving heat fluxes (e.g. fire radiative power) as a function of time along with the consumption and plume rise observations should be attempted, thus providing data sets that can be used for comprehensive model validation and refinement.
Conclusion
We have shown that multi-remote sensor observations of a rotating pyroconvective plume help elucidate the processes leading to plume rotation and towering pyroCu development. To be specific, the interleaving of RHI and PPI scans from two Doppler lidars and a polarimetric Ka-band radar during a stand-replacing prescribed fire reveal the following:
During the early phases of the fire, a narrow and upright ‘starting plume’ rising from an isolated fire heat source developed. This plume did not produce significant flow modification far from the plume base and only yielded shallow pyroCu.
In contrast, during the fire’s second phase, strong anticyclonic whole-column plume rotation (±15 m s−1) developed. The plume rotation developed contemporaneously to the indrafting of the initial plume into a newly developing plume linked to secondary helitorch ignitions. Although the observed rotation is sub-tornadic in the present case, it nevertheless yielded an order of magnitude increase in the winds impacting the fire.
During the fire’s third phase, towering pyroCu development with cloud tops reaching 10 km occurred. The towering pyroCu (borderline pyroCb) possessed an archetypal central updraft and flanking downdraft structure with sub-cloud and in-cloud updrafts of ~35 m s−1. These observations reinforce our understanding of the extreme updrafts associated with these fire-generated clouds linked to deep convective columns.
The fuel consumption data suggest the deepest plume cores and strongest updrafts were linked to a spatially expansive region of large consumption values, and thus large inferred sensible heat fluxes.
Although these observations help us understand links between plume dynamics and fire processes in this case, there are two deficits in this study: first, the lack of contemporaneous detailed fire sensible flux measurements precludes our ability to evaluate temporal covariations between plume dynamics and fire processes. For example, although it appeared (by eye) that the onset of plume rotation led to increased combustion rates, we cannot quantitatively assess lead–lag relationships or feedbacks between these processes or disentangle the contribution of locally higher fuel loads or local flow–topography interaction. Second, the lack of proximal thermodynamic and wind profile data, coupled with the absence of fire heat fluxes make it difficult to fully understand the processes leading to the observed pyroconvection. Lastly, we also note that our present analysis provides case-specific details of plume and fire-generated winds during a prescribed fire. The broader applicability of these insights will need to be examined in the context of previous and, hopefully, future observations spanning a wider set of fire and environmental conditions. Future experiments that can accomplish such measurements with airborne infrared camera systems and proximal radiosondes (or unmanned aerial systems) would be particularly valuable when paired with plume observations from radars and lidars.
Data availability statement
The raw radar and lidar data used in this study are available: Neil Lareau. (2023). UNR Doppler lidar data (data set). Zenodo. https://doi.org/10.5281/zenodo.7779234. Neil Lareau. (2023). SJSU Doppler Lidar Data (data set). Zenodo. https://doi.org/10.5281/zenodo.7779188. The SJSU Ka-band radar data is available on request at https://www.fireweather.org/data-request.
Conflicts of interest
Craig Clements and Andrew Hudak are Associate Editors of the International Journal of Wildland Fire. To mitigate this potential conflict of interest, they were blinded from the peer review process and were not involved at any stage in the editing of this manuscript.
Declaration of funding
Funding for the radar and lidar plume observations and analysis was provided by the National Science Foundation’s Physical and Dynamic Meteorology Program under Awards AGS-2114251, AGS-1727052 and AGS-1807774 and the USFS Pacific Northwest Research Station under Contracts 19-CR-11261987-062 and 18-CR-11261987-085. Funding for the field plot and ALS data collections to measure and map fuels was provided by the Joint Fire Science Program’s Fire and Smoke Model Evaluation Experiment (FASMEE; Project 15-S-01-01) and the USFS Rocky Mountain Research Station (RMRS). Fuels data analysis was funded via Joint Venture Agreements 17-JV-166 and 19-JV-165 between RMRS and the University of Idaho.
Author contributions
N. L.: lead analysis, writing, figures, and deployed UNR Doppler lidar. C. C.: experiment design lead, SJSU radar and lidar deployment. A. H. and R. M. performed the fuel consumption analysis. A. K.: experiment design support, SJSU radar and lidar deployment. T. A.: SJSU radar operations. R. O.: project planning, fuel surveys and photographs.
Acknowledgements
We thank the Fishlake National Forest for conducting the prescribed fire and allowing research crews access to these fires. We also thank Holt Hanley and Matthew Brewer for their assistance in setting up instrumentation. This research was supported by the U.SDA Forest Service Rocky Mountain Research Station. The findings and conclusions in this publication are those of the authors and should not be construed to represent any official USDA or U.S. Government determination or policy.
References
Aydell TB, Clements CB (2021) Mobile Ka-band polarimetric Doppler radar observations of wildfire smoke plumes. Monthly Weather Review 149, 1247-1264.
| Crossref | Google Scholar |
Banta RM, Olivier LD, Holloway ET, Kropfli RA, Bartram BW, Cupp RE, Post MJ (1992) Smoke-column observations from two forest fires using Doppler lidar and Doppler radar. Journal of Applied Meteorology and Climatology 31, 1328-1349.
| Crossref | Google Scholar |
Bluestein H B, Weiss CC, Pazmany AL (2004) Doppler radar observations of dust devils in Texas. Monthly Weather Review 132, 209-224.
| Crossref | Google Scholar |
Church CR, Snow JT, Dessens J (1980) Intense atmospheric vortices associated with a 1000 MW fire. Bulletin of the American Meteorological Society 61, 682-694.
| Crossref | Google Scholar |
Clark TL, Jenkins MA, Coen J, Packham D (1996) A coupled atmosphere–fire model: convective feedback on fire-line dynamics. Journal of Applied Meteorology and Climatology 35(6), 875-901.
| Crossref | Google Scholar |
Clements CB, Zhong S, Goodrick S, Li J, Potter BE, Bian X, Heilman WE, Charney JJ, Perna R, Jang M, Lee D, Patel M, Street S, Aumann G (2007) Observing the dynamics of wildland grass fires: FireFlux—a field validation experiment. Bulletin of the American Meteorological Society 88(9), 1369-1382.
| Crossref | Google Scholar |
Clements CB, Lareau NP, Kingsmill DE, Bowers CL, Camacho CP, Bagley R, Davis B (2018) The rapid deployments to wildfires experiment (RaDFIRE): Observations from the fire zone. Bulletin of the American Meteorological Society 99, 2539-2559.
| Crossref | Google Scholar |
Clements CB, Kochanski AK, Seto D, Davis B, Camacho C, Lareau NP, Contezac J, Restaino J, Heilman WE, Krueger SK, Butler B, Ottmar RD, Vihnanek R, Flynn J, Filippi J-B, Barboni T, Hall DE, Mandel J, Jenkins MA, O'Brien J, Hornsby B, Teske C (2019) The FireFlux II experiment: a model-guided field experiment to improve understanding of fire–atmosphere interactions and fire spread. International Journal of Wildland Fire 28(4), 308.
| Crossref | Google Scholar |
Coen J, Mahalingam S, Daily J (2004) Infrared imagery of crown-fire dynamics during FROSTFIRE. Journal of Applied Meteorology 43, 1241-1259.
| Crossref | Google Scholar |
Coen JL, Stavros EN, Fites-Kaufman JA (2018) Deconstructing the King megafire. Ecological Applications 28, 1565-1580.
| Crossref | Google Scholar | PubMed |
Dowdy AJ, Fromm MD, McCarthy N (2017) Pyrocumulonimbus lightning and fire ignition on Black Saturday in southeast Australia. Journal of Geophysical Research: Atmospheres 122, 7342-7354.
| Crossref | Google Scholar |
Dozier J (1981) A method for satellite identification of surface temperature fields of subpixel resolution. Remote Sensing of Environment 11, 221-229.
| Crossref | Google Scholar |
Finney MA, McAllister SS (2011) A review of fire interactions and mass fires. Journal of Combustion 2011, 1-14.
| Crossref | Google Scholar |
Forthofer JM, Goodrick SL (2011) Review of vortices in wildland fire. Journal of Combustion 2011, 1-14.
| Crossref | Google Scholar |
Fric TF, Roshko A (1994) Vortical structure in the wake of a transverse jet. Journal of Fluid Mechanics 279, 1-47.
| Crossref | Google Scholar |
Fromm M, Tupper A, Rosenfeld D, Servranckx R, McRae R (2006) Violent pyro‐convective storm devastates Australia’s capital and pollutes the stratosphere. Geophysical Research Letters 33, L05815.
| Crossref | Google Scholar |
Fromm M, Lindsey DT, Servranckx R, Yue G, Trickl T, Sica R, Doucet P, Godin‐Beekmann S (2010) The untold story of pyrocumulonimbus. Bulletin of the American Meteorological Society 91, 1193-1210.
| Crossref | Google Scholar |
Fromm M, Servranckx R, Stocks BJ, Peterson DA (2022) Understanding the critical elements of the pyrocumulonimbus storm sparked by high-intensity wildland fire. Communications Earth & Environment 3, 243.
| Crossref | Google Scholar |
Gibbs J (2016) A skill assessment of techniques for real-time diagnosis and short-term prediction of tornado intensity using the WSR-88D. Journal of Operational Meteorology 4, 170-181.
| Crossref | Google Scholar |
Heus T, Jonker HJJ (2008) Subsiding shells around shallow cumulus clouds. Journal of the Atmospheric Sciences 65, 1003-1018.
| Crossref | Google Scholar |
Jones T, Ahmadov R, James E, Pereira G, Freitas S, Grell G (2022) Prototype of a Warn-on-Forecast System for Smoke (WoFS-Smoke). Weather and Forecasting 37(7), 1191-1209.
| Crossref | Google Scholar |
Kepert JD, Thurston W, Tory KJ (2024) A fast, physically based model of firebrand transport by bushfire plumes. Agricultural and Forest Meteorology 345, 109839.
| Crossref | Google Scholar |
Kingsmill DE, French JR, Lareau NP (2023) In situ microphysics observations of intense pyroconvection from a large wildfire. Atmospheric Chemistry and Physics 23, 1-21.
| Crossref | Google Scholar |
Lang TJ, Rutledge SA, Dolan B, Krehbiel P, Rison W, Lindsey DT (2014) Lightning in wildfire smoke plumes observed in Colorado during summer 2012. Monthly Weather Review 142, 489-507.
| Crossref | Google Scholar |
Lareau NP, Clements CB (2016) Environmental controls on pyrocumulus and pyrocumulonimbus initiation and development. Atmospheric Chemistry and Physics 16, 4005-4022.
| Crossref | Google Scholar |
Lareau NP, Clements CB (2017) The mean and turbulent properties of a wildfire convective plume. Journal of Applied Meteorology and Climatology 56, 2289-2299.
| Crossref | Google Scholar |
Lareau NP, Nauslar NJ, Abatzoglou JT (2018) The Carr Fire vortex: a case of pyrotornadogenesis? Geophysical Research Letters 45, 13-107.
| Crossref | Google Scholar |
Lareau NP, Nauslar NJ, Bentley E, Roberts M, Emmerson S, Brong B, Mehle M, Wallman J (2022) Fire-generated tornadic vortices. Bulletin of the American Meteorological Society 103, E1296-E1320.
| Crossref | Google Scholar |
Lareau N (2023) UNR Doppler lidar data [Data set]. Zenodo. doi:10.5281/zenodo.7779234
LaRoche KT, Lang TJ (2017) Observations of ash, ice, and lightning within pyrocumulus clouds using polarimetric NEXRAD radars and the National Lightning Detection Network. Monthly Weather Review 145, 4899-4910.
| Crossref | Google Scholar |
Lee JM, Mirocha JD, Lareau NP, Whitney T, To W, Kochanski A, Lassman W (2023) Sensitivity of Pyrocumulus Convection to Tree Mortality During the 2020 Creek Fire in California. Geophysical Research Letters 50(16), e2023GL104193.
| Crossref | Google Scholar |
Lindley T, Anderson AR, Mahale VN, Curl TS, Line WE, Lindstrom SS, Bachmeier AS (2016) Wildfire detection notifications for impact-based decision support services in Oklahoma using geostationary super rapid scan satellite imagery. Journal of Operational Meteorology 4, 182-191.
| Crossref | Google Scholar |
Lindley TT, Zwink AB, Gravelle CM, Schmidt CC, Palmer CK, Rowe ST, Heffernan R, Driscoll N, Kent GM (2020) Ground-based corroboration of GOES-17 fire detection capabilities during ignition of the Kincade Fire. Journal of Operational Meteorology 8, 105-110.
| Crossref | Google Scholar |
Luderer G, Trentmann J, Andreae MO (2009) A new look at the role of fire-released moisture on the dynamics of atmospheric pyro-convection. International Journal of Wildland Fire 18, 554-562.
| Crossref | Google Scholar |
Marquis J, Richardson Y, Wurman J, Markowski P (2008) Single-and dual-Doppler analysis of a tornadic vortex and surrounding storm-scale flow in the Crowell, Texas, supercell of 30 April 2000. Monthly Weather Review 136, 5017-5043.
| Crossref | Google Scholar |
McCarley TR, Hudak AT, Sparks AM, Vaillant NM, Meddens AJH, Trader L, Mauro F, Kreitler J, Boschetti L (2020) Estimating wildfire fuel consumption with multitemporal airborne laser scanning data and demonstrating linkage with MODIS-derived fire radiative energy. Remote Sensing of Environment 251, 112114.
| Crossref | Google Scholar |
McCarley TR, Hudak AT, Restaino JC, Billmire M, French NHF, Ottmar RD, Hass B, Zarzana K, Goulden T, Volkamer R (2022) A comparison of multitemporal airborne laser scanning data and the Fuel Characteristics Classification System for estimating fuel load and consumption. Journal of Geophysical Research: Biogeosciences 127, e2021JG006733.
| Crossref | Google Scholar |
McCarthy N, McGowan H, Guyot A, Dowdy A (2018) Mobile X-pol radar: a new tool for investigating pyroconvection and associated wildfire meteorology. Bulletin of the American Meteorological Society 99, 1177-1195.
| Crossref | Google Scholar |
McCarthy N, Guyot A, Dowdy A, McGowan H (2019) Wildfire and weather radar: a review. Journal of Geophysical Research: Atmospheres 124, 266-286.
| Crossref | Google Scholar |
McRae RHD, Sharples JJ, Wilkes SR, Walker A (2013) An Australian pyro-tornadogenesis event. Natural Hazards 65, 1801-1811.
| Crossref | Google Scholar |
Morrison H, Peters JM, Varble AC, Hannah WM, Giangrande SE (2020) Thermal chains and entrainment in cumulus updrafts. Part I: Theoretical description. Journal of the Atmospheric Sciences 77, 3637-3660.
| Crossref | Google Scholar |
Peterson DA, Fromm MD, Solbrig JE, Hyer EJ, Surratt ML, Campbell JR (2017a) Detection and inventory of intense pyroconvection in western North America using GOES-15 daytime infrared data. Journal of Applied Meteorology and Climatology 56, 471-493.
| Crossref | Google Scholar |
Peterson DA, Hyer EJ, Campbell JR, Solbrig JE, Fromm MD (2017b) A conceptual model for development of intense pyrocumulonimbus in western North America. Monthly Weather Review 145, 2235-2255.
| Crossref | Google Scholar |
Peterson DA, Campbell JR, Hyer EJ, Fromm MD, Kablick GP, Cossuth JH, DeLand MT (2018) Wildfire-driven thunderstorms cause a volcano-like stratospheric injection of smoke. NPJ Climate and Atmospheric Science 1, 1-8.
| Crossref | Google Scholar | PubMed |
Peterson DA, Thapa LH, Saide PE, Soja AJ, Gargulinski EM, Hyer EJ, et al. (2022) Measurements from inside a thunderstorm driven by wildfire: the 2019 FIREX-AQ field experiment. Bulletin of the American Meteorological Society 103, E2140-E2167.
| Crossref | Google Scholar |
Prichard S, Larkin NS, Ottmar R, French N, Baker K, Brown T, Clements C, Dickinson M, Hudak A, Kochanski A, Linn R, Liu Y, Potter B, Mell W, Tanzer D, Urbanski S, Watts A (2019) The fire and smoke model evaluation experiment—a plan for integrated, large fire–atmosphere field campaigns. Atmosphere 10, 66.
| Crossref | Google Scholar | PubMed |
Rasmussen EN, Straka JM, Davies-Jones R, Doswell III CA, Carr FH, Eilts MD, MacGorman DR (1994) Verification of the origins of rotation in tornadoes experiment: VORTEX. Bulletin of the American Meteorological Society 75, 995-1006.
| Crossref | Google Scholar |
Roberts M, Lareau N, Juliano TW, Shamsaei K, Ebrahimian H, Kosovic B (2024) Sensitivity of Simulated Fire-Generated Circulations to Fuel Characteristics During Large Wildfires. Journal of Geophysical Research: Atmospheres In Press.
| Google Scholar |
Rodriguez B, Lareau NP, Kingsmill DE, Clements CB (2020) Extreme pyroconvective updrafts during a megafire. Geophysical Research Letters 47, e2020GL089001.
| Crossref | Google Scholar |
Shamsaei K, Juliano TW, Roberts M, Ebrahimian H, Kosovic B, Lareau NP, Taciroglu E (2023) Coupled fire-atmosphere simulation of the 2018 Camp Fire using WRF-Fire. International Journal of Wildland Fire 32(2), 195-221.
| Crossref | Google Scholar |
Smith BT, Thompson RL, Speheger DA, Dean AR, Karstens CD, Anderson-Frey AK (2020) WSR-88D tornado intensity estimates. Part I: Real-time probabilities of peak tornado wind speeds. Weather Forecasting 35, 2479-2492.
| Crossref | Google Scholar |
Tohidi A, Gollner MJ, Xiao H (2018) Fire whirls. Annual Review of Fluid Mechanics 50(1), 187-213.
| Crossref | Google Scholar |
Tory KJ, Kepert JD (2021) Pyrocumulonimbus firepower threshold: assessing the atmospheric potential for pyroCb. Weather and Forecasting 36, 439-456.
| Crossref | Google Scholar |
Tory KJ, Thurston W, Kepert JD (2018) Thermodynamics of pyrocumulus: a conceptual study. Monthly Weather Review 146, 2579-2598.
| Crossref | Google Scholar |
Turner JS (1962) The ‘starting plume’ in neutral surroundings. Journal of Fluid Mechanics 13(3), 356-368.
| Crossref | Google Scholar |
World Meteorological Organization 2023, Dec 1 Flammagenitus. https://cloudatlas.wmo.int/en/flammagenitus.html
Wurman J, Dowell D, Richardson Y, Markowski P, Rasmussen E, Burgess D, Wicker L, Bluestein HB (2012) The second verification of the origins of rotation in tornadoes experiment: VORTEX2. Bulletin of the American Meteorological Society 93, 1147-1170.
| Crossref | Google Scholar |
Appendix 1.
The temperature of the plume and the thermodynamic environment can be inferred from the nearest radiosonde data, which are from Grand Junction, CO, at the nominal time of 00:00 UTC on 8 November 2019. Note that Grand Junction is ~300 km to the east of the fire, making the representativeness of these data questionable. Fig. A1 shows the temperature and dewpoint temperature in a skew-T, log-P diagram along with an estimated parcel ascent pathway from the lidar observed condensation level at ~5.5 km MSL. Broadly speaking, the environment can be characterised as (1) very dry with large dew point depressions, (2) possessing a quasi-well mixed layer from the surface to ~600 hPa, (3) exhibiting a region of enhanced stability from 510–600 hPa, and (4) an upper troposphere supportive of ‘tall skinny’ convective available potential energy (CAPE) wherein the moist adiabatic ascent (blue line) from the lidar-estimated condensation level (blue dashed line) is marginally warmer than the environment up to an equilibrium level near 10 km, and approximately coincident with the radar observed plume top (black dashed line).
Radiosonde observation from Grand Junction, CO, which is ~300 km east of the fire. This skew-T, log-P diagram shows the vertical variation of temperature (orange) and dew point temperature (green), along with an estimated moist adiabatic ascent from the lidar-observed plume condensation level (blue line). The height of the maximum radar echo top is shown as a black dashed line.
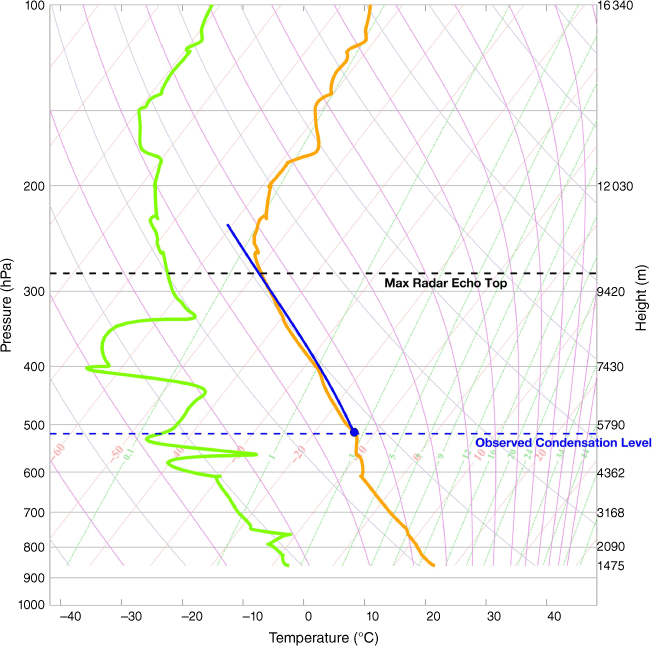