Nanomedicine-based modulation of redox status for cancer therapy
Ping Jin A # , Lei Li B # , Edouard Collins Nice C * and Canhua Huang
A State Key Laboratory of Biotherapy and Cancer Center, West China Hospital, West China School of Basic Medical Sciences and Forensic Medicine, Sichuan University and Collaborative Innovation Center for Biotherapy, Chengdu, 610041, P. R. China.
B School of Basic Medical Sciences, Chengdu University of Traditional Chinese Medicine, Chengdu, 610075, China.
C Department of Biochemistry and Molecular Biology, Monash University, Clayton, Vic. 3800, Australia.
Handling Editor: Mibel Aguilar
Australian Journal of Chemistry 76(8) 337-350 https://doi.org/10.1071/CH22246
Submitted: 24 November 2022 Accepted: 20 February 2023 Published: 2 May 2023
© 2023 The Author(s) (or their employer(s)). Published by CSIRO Publishing.
Abstract
Cancer has always been a major disease with an unfavorable impact on human health worldwide. Redox biology has a close and complicated relationship to the initiation and progression of cancer. Continuous work is being conducted to develop novel approaches for cancer prevention and therapy by modulating redox homeostasis, but problems in drug targeting, drug resistance, adverse effects and recurrence are persistent challenges. Nanotechnology is emerging as a powerful tool to achieve specific targeting, non-invasive therapeutics, high therapeutic efficiency and improved drug sensitivity for cancers by exploiting the features of their microenvironment, especially the redox properties. In addition, nanoplatform-mediated delivery of anticancer drugs or exogenous antioxidants/oxidants affords a promising prospect for cancer therapy. In this review, we will summarize recent advances in redox species-responsive nanoplatforms for tumor treatment. Current nanocarrier mediated strategies that manage redox status for cancer treatment will also be discussed.
Keywords: antioxidants, chemodynamic therapy (CDT), GSH‐responsive nanoplatforms, H2O2‐responsive theranostics, nanotechnology, redox, tumor, tumor microenvironment.
Introduction
Reactive oxidants, such as reactive oxygen species (ROS) and reactive nitrogen species (RNS), become abnormally high and cause oxidative stress.[1] Superoxide (O2˙−), hydroxyl (OH˙−), alkoxyl (RO˙−) and peroxyl (ROO˙−) radicals are unpaired ROS that have higher reactivity since they can either give an electron or accept one from other molecules to reach stability.[2,3] Either endogenous or external factors can produce them. Endoplasmic reticulum (ER), peroxisomes and mitochondria are among the high oxygen-consumption organelles that are the main sources of endogenous ROS.[4] Other endogenous sources of ROS derived from reduced riboflavin include reduced flavin mononucleotide (FMNH2), reduced flavin adenine dinucleotide (FADH2), cytochrome P450 (CYP), monoamine oxidase, xanthine oxidase, cyclooxygenase (COX), glycolate oxidase, hydroxylate oxidase, aldehyde oxidase and amino acid oxidase.[5] Exogenous ROS sources include radiation, heavy metals, transition metals, narcotics, alcohol, tobacco smoke, pollution and industrial solvents. Nitric oxide (NO) and its peroxide derivative are components of RNS (OONO).[6]
Oxidants are counteracted by a robust antioxidant system which consists of antioxidant transcription factors, non-enzymatic reducing power and enzymatic antioxidants. Antioxidant transcription factors include nuclear factor erythroid-derived 2-like 2 (NRF2), forehead box class O (FOXO), activator protein 1 (AP-1), hypoxia-induce factor-1α (HIF-1α) and p53.[7] Non-enzymatic small molecules that can directly remove ROS include glutathione (GSH), bilirubin, α‐tocopherol, ascorbate, CoQ, uric acid, α-lipoic acid, carotenoids and exogenous β-carotene, vitamin E, vitamin C and plant polyphenols. Enzymatic antioxidants include superoxide dismutase (SOD) for scavenging O2˙−, catalase (CAT) for converting H2O2 into H2O and O2, peroxiredoxins (Prxs) for reducing H2O2 to H2O, glutathione peroxidases (GPXs), glutathione reductase (GR), thioredoxins (Trxs) and glutaredoxins (Grxs).[8] Importantly, cellular redox pairings (NAD+/NADH, NADP+/NADPH and GSH/GSSG) ensure the transfer of cellular electrons by serving as coenzymes or substrates for these non-enzymatic and enzymatic systems.[9] In addition, non-classical antioxidant systems exist to cooperatively maintain the cellular redox homeostasis, which are mainly composed of metabolic enzymes, metabolites and antioxidant ‘sacrificial’ proteins, metalloproteins and other signaling proteins.[10]
All aspects of cancer are associated with redox biology. Non-transformed cells have low levels of ROS which act as intracellular signaling molecules responsible for maintaining signal transmission, the inflammatory response and autophagy.[1] Imbalance of redox homeostasis may drive abnormal cellular behaviour and promote the occurrence of cancer. Events such as DNA damage, oncogene activation and loss of tumor suppressor function lead to dysfunction of mitochondria, metabolism and hypoxia, which will further promote ROS production, resulting in higher concentrations of ROS in tumor cells than in normal cells.[11] Cancer cells develop a sophisticated ROS-scavenging machinery at the same time, which gives them a proliferative advantage but also leaves them vulnerable to excessive ROS.[12] On the one hand, adequate ROS levels can trigger the growth factor pathway, the mitogen-activated protein kinase (MAPK) pathway, the phosphatidylinositide 3-kinase/protein kinase B (PI3K/AKT) pathway, the nuclear factor kappa-B (Nf-κb) and the hypoxia inducible factor-1 (HIF-1) pathway, all of which are pro-tumor signaling pathways that could further promote the occurrence, development and metastasis of tumors. On the other hand, when ROS levels exceed the threshold that tumor cells can tolerate, related mechanisms will be triggered to destroy involved biomacromolecules including DNA and oxidized lipids, and eventually lead to apoptosis and necrosis.[13] Taken together, oxidative stress can bidirectionally activate the mechanism of cell proliferation and apoptosis depending on the degree of ROS excess and exposure time. Based on the dual role of ROS in cancer development, antioxidant and pro-oxidative strategies have attracted much attention in the development of antitumor strategies.[14]
Precise targeting of drugs to specific intracellular receptors or organelles unquestionably improves the therapeutic effect. Nanoparticles (NPs) are employed to delivery agents to achieve binding to targeted molecules and release compounds at the right time and right dosage, therefore achieving greater safety, high efficacy and low side effects.[15,16] The design of the wide range of nanosystems currently available is made according to the physiopathology and tumor microenvironment (TME) properties, such as vascular anomalies, oxygenation, pH, perfusion and metabolic states.[17] The advantages of nanomedicine over conventional platforms are as follows: (1) Nanomedicines can easily diffuse or absorb into the body due to their small size; (2) Specialized NPs can target specific TME components; (3) They concentrate in cancerous tissue as opposed to healthy cells as a result of enhanced permeability and retention (EPR) effects, abnormal lymphatic drainage and leaky vasculature; (4) They exhibit prolonged drug retention time and sustained or stimulus-triggered drug release. Notably, nanomedicine has been extensively applied to transforming the immunosuppressive TME into an immunosupportive environment thus re-sensitizing tumor cells to immunotherapy. Intriguingly, high levels of redox species in the TME are used as activators for theragnostic nanoplatforms.[18] Accordingly, redox‐active nanomedicine such as H2O2 and GSH-responsive nanoplatforms have been exploited for selective and effective cancer treatment.[19] On the other hand, multiple NPs that function by neutralizing pro-tumor ROS or by augmenting intracellular oxidative stress have been documented to have outstanding anti-tumor efficiency.[20] Those NPs that elicit ROS levels beyond the threshold alone or simultaneously boost ROS production and deplete GSH, could lead to restructuring of the DNA architecture, inhibition of enzymes and activation of apoptosis.[21] Furthermore, there is growing interest in the idea of employing nanomaterials with inherent redox capacities or assembling complexes with medications or oxidants/antioxidants to obtain redox qualities for the purpose of eradicating malignancies.[22]
Here, we cover the most recent developments in the use of smart nanoplatforms that respond to redox species for effective cancer detection and treatment. Next, emerging strategies based on NPs for effective tumor elimination by modulating intracellular oxidative stress are discussed, with emphasis on ROS boosting, GSH depletion and antioxidants/oxidants delivery systems.
Redox species-responsive tumor-specific delivery nanoplatforms
The stable and robust characteristics of the TME including hypoxia, acidic nature, abnormal vasculature, high level of redox species and overexpressed enzymes make it an alternative target for cancer therapy.[23] A number of smart nanoplatforms have been created by using these characteristics as activators to produce accurate cancer targeted therapy, real-time imaging and effective cancer detection.[24] For instance, hypoxic TME offers the opportunity to use hypoxia-responsive nanoparticles for effective medication delivery to tumors, and a significant amount of data has demonstrated their great anti-tumor efficacy.[25] In addition, pH-responsive nanomaterials possess an overwhelming advantage for controlling controlled drug release.[26] Importantly, redox species are also well-recognized activators, exemplified by the development of H2O2 or/and GSH‐responsive nanoplatforms (Fig. 1).
Redox species-responsive tumor-specific delivery nanoplatforms. Redox species have been exploited as activators to develop a series of smart nanoplatforms to produce in situ O2 or other toxic ROS generation, bioimaging, cargo release and prodrug activation for efficient cancer diagnosis and treatment.
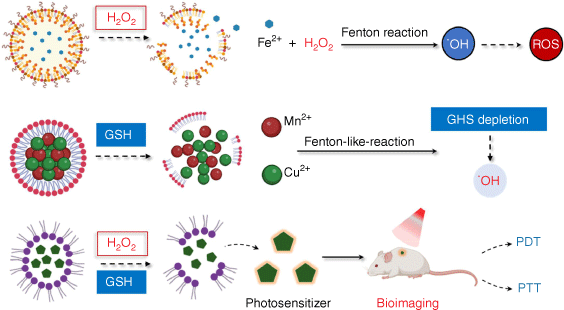
Redox species-responsive nanoplatforms for O2 or other toxic ROS generation
Considered as a hallmark of malignancies, H2O2 which has high stability in the TME, has been employed as a stimulus in nanomaterial drug delivery systems. Meanwhile, GSH at high concentrations maintains the redox balance and can be used in the design of GSH-responsive nanoplatforms for cancer therapy. H2O2 not only functions as a signaling molecule to mediate cellular movements, but also can be decomposed to in situ O2 or other toxic ROS under certain conditions, thus enhancing therapeutic efficacy. Depletion of GSH plays a significant role in redox stress elevation, relying on the Fenton reaction, due to its main function of scavenging ROS.[27] Additionally, redox species-sensitive nanoparticles cannot only achieve drug delivery but also offer bioimaging applications. In addition, many nanoparticles have been designed to respond to redox species to activate their function or release incorporated drugs.
To date, multiple nanoplatforms have been designed to decompose H2O2 to O2 for alleviating hypoxia in the TME or converting H2O2 into highly toxic ˙OH or 1O2 for excessive oxidative stress. An H2O2-responsive degradable nanoplatform (MDSP NP) was created, for instance, by co-loading doxorubicin (DOX), aza-BODIPY (SAB) and a photosensitizer (PS) into MnO2 NPs with a hydrangea structure.[28] In this system, MnO2 can produce oxygen and alleviate tumor hypoxia by reacting with H2O2 and H+ in the TME. The in vitro and in vivo data showed that MDSP NPs could be paired with laser radiation to produce an outstanding anti-tumor impact that was synergistic with chemo/photodynamic/photothermal treatment. Moreover, TME facilitates the generation of highly toxic ˙OH converted from H2O2 in the presence of metal ions such as ferrous (Fe2+) and cupric (Cu2+) due to its mild acidity and overproduced H2O2. Fenton or Fenton-like reactions are what these are called. By using a hydroxide ion to coordinate H2O2 to Cu2+, Lin et al. created a copper peroxide (CP) nanodot, for instance.[29] The release of Fenton catalytic Cu2+ and H2O2 as well as a Fenton-type reaction for the conversion of H2O2 into extremely poisonous ˙OH were both enhanced by the acidic environment of endo/lysosomes in tumor cells. The resultant ˙OH caused cell death via a lysosome-associated route by inducing lysosomal membrane permeabilization by lipid peroxidation. With the development of H2O2 responsive-nanoplatforms or nanomaterials with CAT mimicking activity, multiple antitumor strategies that require the presence of O2 including photodynamic therapy (PDT), chemodynamical therapy (CDT) and sonodynamic therapy (SDT) have achieved better antitumor effects (the mechanism and effect of their action are described in detail below). Moreover, the generation of O2 in situ by catalyzing H2O2 could overcome tumor hypoxia-associated drug resistance.
Developing nanoagents that can efficiently break redox homeostasis by reducing tumor GSH levels has also emerged as an efficient tumor therapy. Generally, multivalent metal ions such as Fe3+/Fe2+, Cu2+/Cu+, Mn4+/Mn2+ and Co3+/Co2+ exhibit GSH-responsive via a valence shift.[30] An illustration is a novel albumin-based multifunctional nanoagent for GSH-depletion that was created by co-growing CuO and MnOX inside of albumin molecules and then conjugating the resulting nanoagent with a PtIV prodrug. Copper species can produce ˙OH in weakly acidic conditions (pH = 6.5), whereas MnOX can interact with GSH and deplete it, reducing the synthesis of GSH-Pt adducts and ˙OH consumption. Thus, this system can inhibit GPX-4 expression and achieve better chemotherapy and chemodynamic therapy effects.[31] Gong et al. designed a nanomedicine by anchoring gold onto a carbon-dot (CAT-g), followed by conjunction with triphenylphosphine (TPP) and cinnamaldehyde (CA) on its surface to disturb mitochondrial redox homeostasis.[32] After the liberation of CA in response to the acidity of the endosomes, TPP-CAT-g quickly reacted with mitochondrial GSH and elevated ROS production. These data showed that MitoCAT-g treatment significantly inhibits tumor growth in subcutaneous and orthotopic patient-derived xenograft (PDX) hepatocellular carcinoma models, indicating that MitoCAT-g is a promising agent for anticancer applications.
Clearly, oxidative stress might be effectively increased to boost therapeutic effects in cancer treatment by simultaneously increasing ROS levels and depleting GSH. Mesoporous copper/manganese silicate nanospheres (mCMSNs) coated with cancer cell membranes were developed by Liu et al. to convert endogenous H2O2 into O2 and then react with O2 to create harmful 1O2.[33] While this is going on, Cu+, Mn2+ and ˙OH can be produced by the Fenton-like reaction that is catalyzed by the GSH-triggered degradation of mCMSNs. They showed that by converting endogenous H2O2 into O2 and then reacting with O2 to produce harmful 1O2 when exposed to a 635 nm laser, mCMSNs might alleviate the tumor hypoxic microenvironment. For effective hydroxyl radical (˙OH) formation, GSH-triggered mCMSNs biodegradation can simultaneously produce Fenton-like Cu+ and Mn2+ ions and deplete GSH. This simultaneous hypoxia relieving and GSH depletion system disrupts the cellular antioxidant defense system, thus exhibiting exceptional anti-tumor therapeutic effects. Through the coprecipitation of upconversion nanoparticles (UCNPs) and AIE-active photosensitizers, followed by the formation of MnO2 as the outer shell, Wang et al. created a GSH-depleting and near-infrared (NIR)-regulated nanoplatform.[34] UCNPs in this system cause the activation of AIE-active photosensitizers to produce ˙OH under exposure to NIR irradiation. The MnO2 shell was decomposed to Mn2+ by intracellular GSH, which efficiently depletes GSH and elevates intracellular ˙OH. Notably, the resulting Mn2+ was subsequently capable of catalyzing intracellular H2O2 to ˙OH. As a result, tumor growth was significantly inhibited by this triple-jump ˙OH generation protocol with no noticeable side effects.
Redox species-responsive nanoplatforms for bioimaging
Redox species-sensitive nanoparticles cannot only deliver cytotoxic drugs but also provide bioimaging applications. The bioimaging of H2O2-responsive theranostics is mainly achieved by the quench of H2O2-sensitive fluorescent dyes. Nanomaterials containing metal ions,[35] arylboronic ester[36] and aryl oxalate ester[37] have been reported for bioimaging. An example is the Cu/CC NPs, which are synthesized by assembling a photosensitizer (Chlorine e6, Ce6) and modified carbon dots (CDs-Ce6) and Cu2+ and exhibit quenched fluorescence (FL) imaging and photosensitization. In addition, Cu2+ not only reacts with H2O2 to provide extra chemodynamic therapy (CDT) but also depletes GSH in tumors, thus enhancing the efficacy of ROS based therapy.[38] Recently, photoacoustic imaging (PAI) has emerged as a promising imaging modality for cancer diagnosis due to its good penetration into deep tissue and fine spatial solubility. A graphene quantum dot nanozyme (GQDzyme)/2,2′-azino-bis (ABTS) based exosome-like nanozyme vesicle was developed by Ding et al.[39] In the presence of H2O2, the GQDzyme effectively catalyzes ABTS into its oxidized form, which is an ideal contrast agent for PAI due to its strong NIR absorbance. Their research suggested that this exosome-like nanozyme vesicle, with excellent biocompatibility and blood circulation stealth ability, effectively accumulated and selectively triggered catalytic PAI in nasopharyngeal carcinoma (NPC). To further achieve imaging-guided tumor-targeted effective PDT, Zeng et al. reported a novel H2O2-activatable and biodegradable nanomedicine (BSA-MBPB) that encapsulates pro-photosensitizer (MBPB) into bovine serum albumin (BSA) with its photoactivity completely ablated within BSA.[40] H2O2 activates BSA-MBPB to not only produce the photosensitizer methylene blue for recovering its fluorescent for dual-modal imaging (photoacoustic and photosensitizing) and cytotoxic singlet oxygen (1O2) generation, but also provide adjuvant quinone methide to boost the 1O2 by GSH-depletion.
Studies have also suggested that the redox reaction between nanoplatforms and GSH provides bioimaging applications for diagnosis and treatment of cancer. Multiple imaging modalities with GSH-responsiveness such as MRI, computed tomography, optical imaging and upconversion luminescence (UCL) have been employed in the preclinic and clinic. For instance, He et al. reported on a drug delivery system that targets the mitochondria and is designated as CDs (DOX)@MSN-TPP@AuNPs.[41] This mesosystem worked as a promising fluorescent probe (λex = 633 nm, λem = 650 nm) for targeted imaging of mitochondria using NIR fluorescence to increase therapeutic effectiveness and safety after being etched with GSH. In the meantime, mitochondrial depolarization and subsequent death can be induced in cells by the release of DOX in a GSH-dependent manner. Xu et al. designed a nanoagent based on the inherent UCL/CT/MRI abilities of upconversion nanoparticles (UCNPs) for imaging-guided therapy.[42] By rupturing the brittle Mn-O link and releasing Mn under acidic and high GSH conditions, the MRI effect was achieved. Additionally, this nanoagent was given numerous imaging capabilities to realize imaging-guided cancer therapy thanks to MRI/CT/UCL imaging produced from Gd3+/Yb3+/Nd3+/Er3+ co-doped UCNPs under 808 nm laser excitation. Overall, by combining monitoring tumor uptake and drug liberation on one platform, various imaging modality based nanotheranostic agents are anticipated to perform well in cancer therapy.
Redox species-responsive nanoplatforms for cargo release and prodrug activation
In addition to the above-mentioned applications, redox species can serve as a stimulus to activate the controllable release of drugs from nanosystems.[43] For instance, a ROS-triggered prodrug nanoplatform was created by pyropheophorbide-induced self-assembly of CTX-S-OA or CTX-Se-OA into nanoparticles (PPa).[44] CTX was selectively and rapidly released in response to not only H2O2 in tumor tissue, but also PPa generated ROS under laser irradiation. The tumor distribution and systemic circulation of both CTX and PPa were also greatly prolonged by this prodrug-nano combination, showing promising synergistic therapeutic effects in vivo. Lee et al. have prepared PL-incorporated ChitoPEGse nanoparticles to target pulmonary metastasis of cancer cells by crosslinking selenocystine-acetyl histidine (Ac-histidine) with methoxy poly(ethylene glycol)-grafted chitosan (ChitoPEG) for delivery of piperlongumine (PL, a natural alkaloid).[45] In their investigation, PL showed superior anticancer and antimetastatic efficacy in vitro and in vivo and was released in response to H2O2 and acidic stimulation. Taken together, nanoplatforms containing H2O2-responsive moieties hold promise in stimuli-driven drug release.[46]
Nanoplatforms containing GSH-activated prodrug/cargoes have also been found to improve drug release and chemotherapeutic effectiveness. A pH/reductive dual-sensitive polymeric prodrug (P(OE DS-CP)) was created in 2019 by He et al. through condensation of cisplatin derivatives (PtIV) with an ortho ester monomer.[47] Prodrugs have the potential to self-assemble into micelles, load DOX and then form a synergistic drug delivery system. To release CDDP quickly and completely and DOX into tumor cells, the polymer backbone might be broken down at a low pH in the high GSH TME. In vitro and in vivo studies revealed that the DOX-loaded micelles could be triggered to release two drugs into tumor tissues which exhibited synergistic antitumor effects. Additionally, GSH has the ability to break numerous redox-sensitive chemical bonds, including disulfide bonds (–S–S–), diselenide bonds (–Se–Se–) and carbon–diselenide bonds (–C–Se–), resulting in the disintegration of nanostructures and the targeted release of drugs at the tumor site.[48] For instance, a stimulus-responsive silica nanoparticle (SNP) integrated with a disulfide crosslinker for GSH-responsive cargo release capability incorporating an imidazole-containing component for endosomal escape capability was reported.[49] Multiple cargos such as nucleic acids (e.g. DNA and mRNA) and CRISPR genome editors (e.g. Cas9/sgRNA ribonucleoprotein (RNP), and RNP with donor DNA) can be delivered by this SNP with excellent efficiency and biocompatibility to target cancer cells. Overall, these studies suggest an exciting strategy for specific drug release in the tumor.
Disturbing redox homeostasis by nanoparticles
Suppressing oxidative stress has long been regarded as a promising strategy for cancer therapy due to the crucial role that ROS play in cancer biology. On the other hand, recent research indicates that oxidative damage induction and programmed cell death can also be useful cancer treatment methods.[50] A significant amount of data has demonstrated that development of nanoparticle-based therapy holds huge potential to modulate redox homeostasis for cancer therapy (Fig. 2).
Strategy and application of nanoparticle-mediated redox modulation for cancer treatment. A significant amount of data has demonstrated that development of nanoparticle-based therapy holds huge potential to modulate redox homeostasis for cancer therapy by direct delivery of ROS inhibitors/inducers, natural compounds and enzymes.
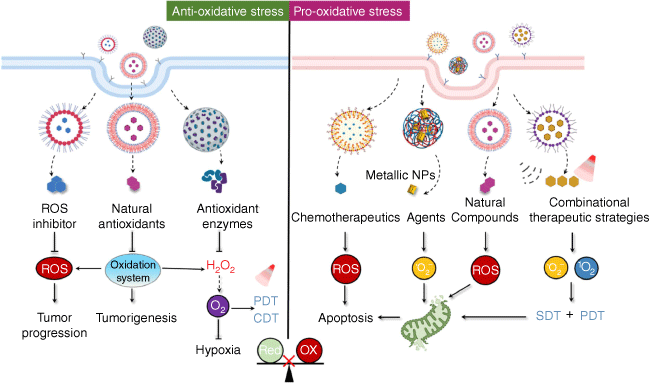
Nanoparticle-mediated suspension of moderate oxidative stress for cancer therapy
By promoting numerous biochemical processes including signal transduction, autophagy and proteolysis, moderate ROS has been shown to assist the physiological functions of various diseases, including cancer. Scavenging pro-tumor ROS in this situation has the potential to lower the risk of cancer and slow the spread of the disease. Obviously, direct delivery of ROS inhibitors such as N-acetyl-l-cysteine (NAC), sodium azide (NaN3) and mannitol, as well as those agents that could inhibit ROS production by targeting related signaling pathways or node molecules possess huge potential to inhibit ROS for tumor suppression, and a plethora of studies have demonstrated their application.[51] Recently, nanocarrier loaded natural antioxidants, antioxidant enzymes or their mimics have attracted huge interest from investigators.
Natural antioxidants such as curcumin, resveratrol, polyphenols and flavonoids have been reported to counteract and neutralize ROS in the tumor or TME thereby inhibiting tumors.[52] Nano-antioxidants, referring to encapsulations of antioxidants into nanocarriers, antioxidant-derived nanoparticles, can achieve safe and efficient delivery of a high payload of antioxidants for eradicating cancer. One phytosterol found in a variety of food-producing plants, such as natural oils, nuts, and soy products, is called sitosterol. It has been shown to have therapeutic potential for decreasing cholesterol and low-density lipoprotein, scavenging free radicals and preventing cancer. Sitosterol-derived nano-antioxidant therapy employing alginate/chitosa nanoparticles (sito-Alg/Ch/NPs) was described by Afzal et al.[53] In vitro and in vivo results showed that these NPs exhibited higher cytotoxicity due to enhanced drug release patterns, improved bioavailability and antioxidant potential in breast cancer. Resveratrol, a type of polyphenol, is well-documented to function as an antioxidant for protecting the body from diseases including cancer. Increasingly studies have demonstrated that resveratrol inhibits cellular events associated with tumorigenesis and progression by inhibiting the activity of COX-1 and COX-2, impeding TPA-induced DNA binding of NF-kB.[54] Shi et al. recently created resveratrol-loaded Zein-SHA (low-molecular-weight sodium hyaluronate) nanoparticles. According to increased ABTS free radical scavenging ability and iron (iii) reduction power, resveratrol in capsules has stronger antioxidant activity than free resveratrol. With IC50 values of 14.73 and 17.84 g mL–1, respectively, this nanosystem also demonstrated more antiproliferative activity than free resveratrol in 4T1 murine breast cancer cells.[55] By encasing antioxidants in nanoparticles, researchers can increase the compounds’ bioavailability, biodistribution and capacity to target specific tissues or receptors, resulting in a regulated release of chemicals over an extended period of time.
Recently, the delivery of antioxidant enzymes or their mimics is emerging as a method of reducing oxidative stress for tumor suppression. Mainstream research has been devoted to efficiently delivering CAT and SOD among numerous enzymes to mitigate oxidative stress for therapeutic purposes because the activity of other enzymes requires many co-factors and/or secondary enzymes for proper function. CAT and its mimics have been widely utilized to convert H2O2 into O2 by taking advantage of the high levels of H2O2 in TME or inside tumors as described above. Recently, Wu et al. introduced a competent nanoplatform (denoted CSI@Ex-A) fabricated by encapsulating catalase (CAT) into silica nanoparticles (CAT@SiO2) and then loading them with sonosensitizer indocyanine green (ICG), and further coating with AS1411 aptamer-modified macrophage exosomes.[56] In this approach, CAT@SiO2 is dedicated to driving tumor hypoxia; the role of the macrophage exosomes is to cross the blood–brain barrier (BBB). After tumor cell endocytosis, this nanoplatform undergoes GSH triggered biodegradation and effective O2 self-supply, thereby emerging as a promising therapeutic modality for glioblastoma (GBM). Additionally, a proliferation of nanomaterials with CAT mimicking action, such as metal–organic frameworks (MOFs), manganese dioxide (MnO2) and cerium oxide (CeO2) nanoparticles, has attracted significant interest in biological applications for the treatment of cancer.[57] Recently, a CAT@PtIV-liposome system was designed by encapsulating catalase inside cisplatin(iv)-prodrug-conjugated phospholipid formed liposome nanoparticles. This system shows outstanding enzyme activity to trigger decomposition of H2O2 into O2 for hypoxia relief. After X-ray radiation, treatment of CAT@PtIV-liposome induces significant DNA damage in cancer cells, with potential for clinical translation.[58] Notably, studies were conducted to simultaneously encapsulate CAT and SOD in wind chime-like cyclodextrin (WCC) for efficient protein delivery.[59] By simply mixing with protein solution to create SC/WCC, the amphiphilic WCC may self-assemble into nanoparticles in aqueous solution and provide better encapsulation of these two antioxidant enzymes. This nanoparticle can integrate the synergistic impact of SOD and CAT to increase the elimination of reactive oxygen species (ROS) thanks to the safe and effective transport provided by WCC nanovehicles.
Nanoparticle-mediated amplification of oxidative stress for cancer therapy
While modest levels of ROS activate multiple pro-tumor signaling pathways, excessive ROS exert cytotoxic effects by oxidizing biomolecules such as nucleic acids and lipids. Consistent with the notion that the ROS levels of tumor cells are elevated compared to their normal counterparts, tumor cells are more vulnerable than non-transformed cells to pharmacological treatments that induce excessive ROS accumulation. In fact, many organic and inorganic substances have the ability to cross the ROS threshold and activate multiple signaling pathways, such as p53, tumor necrosis factor (TNF)-signaling, extracellular signal-regulated kinase (ERK) and p38 mitogen-activated protein kinase (MAPK), leading to oxidative damage and programmed cell death in a number of malignancies.[60] Recent advances in nanotechnology provide novel and powerful tools to specifically target tumors and enhance the performance of active compounds. Various NPs, particularly metallic NPs, can directly interact with the cellular membranes, organelles or macromolecules to induce oxidative stress.[61] In fact, a large body of research has shown that the toxicity of NPs is frequently linked to the creation of oxidative stress, which leads to genomic instability, protein malfunction and eventually deregulates enzyme activity, causing cellular dysfunction and cell death in cancer cells.[62]
Metallic NPs have improved potential to take advantage of the oxidative radicals generated by the metals for selective destruction of diseased cells, thus emerging as valuable and efficient diagnostic and anticancer agents.[63] Generally, metallic elements function as charged vehicles and generate soluble cations to form complexes with natural cellular components.[64] Additionally, metal ions with a positive charge may regulate electron transport to help catalyze substrates and enzymes. According to certain studies, metallic nanoparticles can stimulate inflammatory cells like neutrophils and macrophages to produce more ROS. Certain metals, particularly transition metals, were discovered to promote the production of free radicals and lipid peroxidation (LPO). These metals include titanium dioxide (TiO2), zinc oxide (ZnO), cerium oxide (CeO2) and silver (Ag). For instance, it has been shown that ZnO NPs can produce excessive ROS production and activate mitochondrial-mediated apoptotic signaling, making them useful for anti-cancer research. The polymer-modified zinc peroxide nanoparticles (ZnO2 NPs) that Lin et al. described are noteworthy because they combine endogenous and external ROS to intensify oxidative stress.[65] ZnO2 NPs underwent disintegration after being ingested by tumor cells, leading to the regulated release of H2O2 and Zn2+. Inhibiting the electron transport chain (ETC) could cause an increase in the production of mitochondrial O2˙− and H2O2 which would have an additive effect on the anticancer effects of exogenously produced H2O2. Due to their good chemical stability and strong redox potential, TiO2 and ZnO-based oxides have been most frequently used. In terms of their cytotoxicity, the majority of TiO2 and CeO2 nanomaterials (NMs) were only marginally cytotoxic and had no long-term impact on the effectiveness of colony formation. However, ZnO and Ag NMs had an adverse effect on cell survival and produced significant DNA damage at cytotoxic levels.[66] In a similar vein, another study found that TiO2 nanoparticles at concentrations between 0.1 and 100 g mL–1 did not appear to be toxic to peripheral blood mononuclear cells, whereas ZnO2 nanoparticles caused severe damage at concentrations of 1 g mL–1, inhibited the production of interleukin-1 and interleukin at 6.5 g mL–1 and at higher concentrations promoted aggregation and degradation of malate dihydrogen.[67]
Several nanosystem-based therapeutic strategies that can selectively kill cancer cells by ROS generation have aroused enormous attention due to their advantages such as an excellent safety profile, minimal toxicity and non-invasiveness.[68] The most representative amongst them is photodynamic therapy (PDT), which employs a light excited photosensitizer (PS) to generate ROS in the presence of O2 to selectively kill tumors.[69] In this process, the PS converts from the ground state into a singlet-excited state, and then a triplet-excited state upon absorption of photons to generate ˙O2− or 1O2 by undergoing electron transfer with biomolecules. Obviously, the supply of sufficient quantities and the capability of photosensitizers for ROS production are robust tools for the enhancement of the therapeutic efficacy of PDT. In this sense, the use of biocompatible metallic nanoparticles, AuNPs and AgNPs, is particularly exciting, and numerous studies have shown the successful use of PDT in a variety of cancers, including colorectal, lung, cervical, liver and GBM malignancies.[70] For instance, a study by Dai et al. showed that g-C3N4 nanosheet coated with AuNPs could produce a lot of ROS when exposed to a 670 nm laser, considerably suppressing human non-small cell lung cancer, breast cancer and human cervical carcinoma both in vitro and in vivo.[71] Indeed, PDT has been available for more than 40 years with multiple formulations, including AuNPs, having been investigated in clinical trials[72] (NCT01679470, NCT00848042), and notably, several PSs, such as Photofrin, Foscan and Rada chlorin, have been used in the clinic.[73]
Chemodynamic therapy (CDT) is an emerging strategy that produces toxic ˙OH independent of external light by undergoing a Fenton or Fenton-like reaction in the presence of excessive intracellular H2O2 in tumor tissues.[74] As is well known, ˙OH possesses higher activity than 1O2, thus providing better tumor-killing ability. So far, multiple investigations have demonstrated the promising efficiency of CDT in multiple tumors.[75] Recently, Zhang et al. summarized the development of CDT and their antitumor applications, with a critical discussion about the future development trend and challenges of CDT.[76] Another emerging strategy is sonodynamic therapy (SDT), which utilizes ultrasound to activate agents for ROS generation, thus inducing apoptosis in cancer cells.[77] For instance, by encapsulating the sonosensitizer chlorin e6 (Ce6) in the core and then conjugating anti-PD-L1 antibody (aPD-L1) to the interlayer, with a PEG coating, which could be sheddable at a low pH value (6.5) of the TME, Yang et al. created a lipid (LP)-based micellar nanomedicine.[78] With only mild immune-related side effects, this approach enabled tumor-targeting administration that triggered anti-tumor immunity (irAEs). A SDT activated by ultrasonic insonation also generates tumor-killing ROS in conjunction with immunity, resulting in a potent anti-cancer immunity and long-term immunological memory to successfully inhibit melanoma. Overall, these novel therapies offer fresh ideas for creating integrated anticancer drugs based on NPs that target and eliminate cancerous cells by raising their ROS setpoint with little invasion.
Interestingly, several natural compounds have recently gained significant attention as anti-tumor medicines due to their capacity of eliciting oxidative stress.[79] For instance, excessive doses of polyphenols can have pro-oxidant lethal effects by suppressing the Nrf2 pathway and antioxidative genes like GPX, heme oxygenase-1 (HO-1) and sirtuin-1 from being expressed (Sirt1). As a result, NP-based controlled drug delivery systems that are secure and appropriate have been utilized to assure effective administration of these therapeutic candidates into the tumor site. As an illustration, Shi et al. found that ZnO-PBA-Curcumin, a phenyl boronic acid (PBA)-conjugated and pH-responsive ZnO nanoparticles loaded with Curcumin (a polyphenol obtained from the herb Curcuma longa), can induce apoptotic cell death in MCF-7 human breast cancer cells by inducing oxidative stress and mitochondrial damage.[80] Quercetin, a plant-derived bioflavonoid loaded into PBA-ZnO nanoparticles (referred to as PBA-ZnO-Q), caused apoptosis in MCF via enhancing oxidative stress and mitochondrial damage, as demonstrated by Sadhukhan et al.[81] Together, these significant findings suggest that phytochemicals hold potential to eradicate cancer cells by ROS-induced cell death and apoptosis, the nanoparticles ensuring their efficient transport and high efficacy against tumors.
Discussion
As known, cancer cells undergo metabolic reprogramming to boost ROS production as it is crucial for all aspects of cancer. Meanwhile, they have evolved a set of ROS-scavenging systems to avoid ROS-induced damage. The interaction of these two opposing systems raises the homeostatic ROS setpoint, which gives cancer cells an edge in terms of proliferation.[82] In that context, redox modulation represents a reasonable strategy for the prevention and treatment of cancer. On the one hand, neutralizing moderate ROS that is crucial for carcinogenesis would suppress a tumor by restricting cellular signaling transduction, physiological activity and the synthesis of biomolecules. On the other hand, because there is a larger level of ROS, cancer cells are more susceptible to an increase in ROS that will result in macromolecular harm and cell death.
While it is well demonstrated that parturition of the cellular redox balance provides promising therapy for eliminating cancer cells, their efficiency is limited not only by drug resistance and tumor relapse,[83] but also the properties of the drug itself and the targeting strategy. Most common chemotherapeutic medications frequently fail to eradicate tumors and may even trigger the development of therapy-induced cancers. Notably, selectively killing cancer cells with minimal toxicity and high safety are the primary challenges in cancer therapy. Drugs employed in standard chemotherapy often fail to discriminate between cancer and healthy cells. Additionally, unwanted side effects are common and can occur with any treatment, including those that are explicitly intended to target altered signaling pathways in cancer cells. Even though suitable natural antioxidants/pro-oxidants exhibiting tumoricidal effect alone or in combination with radiotherapy or chemotherapy have been identified, there is still a long way to go before these therapies enter the clinic. Their effectiveness is significantly hampered by drawbacks like non-specific tumor targeting, poor membrane permeability, short blood circulation times, complicated physiological processes and other challenges, and their clinical use for cancer therapy is constrained due to the paucity of conclusive human research data.
These issues can be resolved by the development of nanocarrier systems that efficiently deliver therapeutic candidates into the tumor site with little invasiveness. Redox species can function as essential ‘regulators’ or efficient ‘targets’ for antitumor therapeutics that integrate nanotechnology with redox modulating strategies (Table 1). On the one hand, excessive H2O2 in TME has been used to generate O2 in the presence of CAT or other nanomaterials, with CAT simulating the necessary activity to treat hypoxia and make tumors more responsive to PDT, SDT, chemotherapy, radiation and other therapies. Additionally, H2O2 can be transformed into poisonous forms of OH, 1O2, O2 or RNS (NO), which can harm cancer cells by oxidation. High GSH-responsive theranostics have also been developed for the stepwise release of drugs or biomacromolecules, prodrug delivery and triggering of CDT by integrating GSH cleavable linkers such as S–S, Se–Se, C–Se and Au–S into nanocarriers.[18] Multimodal imaging that responds to H2O2 or GSH is another important use in nanomedicine. On the other hand, nanomedicines have been shown to be effective in cancer therapy by deleting pro-tumor moderate ROS or pushing cancer cells beyond their oxidative stress limits that can destroy them. Notably, nanocarriers coated with natural antioxidants/pro-oxidants are being used to enhance the efficacy of conventional treatments or to treat tumors by modulating redox status. Despite the significant advancements in nanomedicine, there still remain some problems in this area. Multiple biological processes, including NP–protein interactions, blood circulation, tumor tissue penetration and tumor cell internalization, as well as interaction with the TME, are involved in the systemic transport of NPs, which help determine the therapeutic outcome of NP-based treatments. Additionally, NP characteristics such as size, geometry, elasticity, stiffness, composition and targeted ligand might also affect the enhanced permeability and retention (EPR) effect (by which nanomedicine accumulates in the tumor) and the therapeutic results.[84] It should be noted that the ongoing use of multifunctional nanodrugs has been constrained by material restrictions, such as toxicities and inadequate drug loadings. Taken together, this means that the complexity and variety of the malignancies and the characteristics of the NPs necessitate rigorous patient selection to determine which patients will benefit from a particular nanotherapy.[68c] Their use requires a safety assessment based on biocompatibility, release, accumulation and metabolism.
Category | Nanoplatforms | Mechanism of action | Efficacy | Tumor type | Ref |
---|---|---|---|---|---|
H2O2 responsive | MDSP NP | Hypoxia relief; Chemo/photodynamic/photothermal therapy | Reduce resistance to DOX; improve therapeutic efficiency | Colorectal cancer (HTC116) | [28] |
Copper peroxide (CP) nanodot | Fenton-type reaction; ˙OH generation; lipid peroxidation | Cell death; tumor growth inhibition | Glioblastoma multiforme (U87) | [29] | |
BSA-MBPB | Dual-modal imaging and 1O2 generation; PDT | Antitumor without major side effects | Hepatocellular carcinoma (HepG) | [40] | |
PPa@prodrug NPs | ROS generation | Antitumor | Breast cancer (4T1) | [44] | |
ChitoPEGse nanoparticles | Apoptosis/necrosis | Superior anticancer and antimetastatic activity | CT26; A549 | [45] | |
GSH responsive | P6 NPs | ˙OH generation; GSH deletion; apoptosis | Inhibiting the growth of cisplatin-sensitive and -resistant cancer cells | Ovarian cancer (A2780) | [31] |
CDs(DOX)@MSN-TPP@AuNPs | Targeted imaging of mitochondria; apoptosis; membrane depolarization of mitochondria | Tumor inhibition | Hela; MCF-7; 4T1 | [41] | |
UCNCs-DOX | Chemo-photothermal therapy; MRI/CT/UCL imaging | High anticancer efficacy | Cervical cancer (HeLa) | [42] | |
MitoCAT-g | Elevated ROS production | Inhibits tumor growth | Hepatocellular carcinoma (HepG2) | [32] | |
P(OE DS-CP) | Apoptosis | Excellent antitumor effect | Hepatocellular carcinoma (HepG2) | [47] | |
H2O2 and GSH responsive | mCMSNs | 1O2 production; Fenton-like reaction; ˙OH generation; tumor hypoxia relief | Inhibits tumor growth | Breast cancer (MCF-7) | [33] |
MUM NPs | Hypoxia relief; ROS generation; GSH-depleting; PDT; bioimaging | Inhibits cancer cell proliferation and damages tumor vascular tissues | Breast cancer (4T1) | [34] | |
Cu/CC NPs | CDT; GSH deletion; fluorescence (FL) imaging; oxidative stress | Enhanced ROS-relevant therapeutic efficiency; antitumor with negligible adverse effects | Breast cancer (4T1) | [38] | |
GQDzyme/ABTS nanoparticle | Photoacoustic imaging (PAI) | Cancer diagnosis | Nasopharyngeal carcinoma (CNE-2) | [39] | |
Suspending moderate oxidative stress | β-sito-Alg/Ch/NPs | Scavenging free radicals | Cytotoxicity | Breast cancer (MCF-7) | [53] |
Resveratrol (RES) loaded Zein-SHA | Antioxidant activity | Antiproliferative activity | Breast cancer (4T1) | [55] | |
CSI@Ex-A | Hypoxia relief; SDT; antioxidant activity | Antitumor | Glioblastoma multiforme (U87) | [56] | |
CAT@Pt (IV)-liposome | Hypoxia relief; DNA damage | Enhanced chemo-radiotherapy of cancer | Breast cancer (4T1) | [58] | |
Amplifying oxidative stress | ZnO2 NPs | ROS production; oxidative damage | Killing cancer cell | Glioblastoma multiforme (U87) | [85] |
g-C3N4 nanosheet | ROS generation | Cancer cell-killing and tumor growth-suppressing | A549; MCF-7; and HeLa | [71] | |
P-aPD-L1/C | Anti-tumor immunity activation; ROS generation; activation of cytotoxic T cells | Suppress melanoma growth and postoperative recurrence | Melanoma (B16–F10) | [78] | |
ZnO-PBA-Curcumin | Inducing oxidative stress and mitochondrial damage | Cell death | Breast cancer (MCF-7) | [80] | |
PBA-ZnO-Q | Oxidative stress and mitochondrial damage | Apoptotic cell death | Breast cancer cells (MCF-7) | [81] |
Overall, modulating redox status by nanoparticles shows enormous potential in cancer therapy (Table 2). To expand their applicability in therapeutic settings, more study is required. The advancement of antioxidant therapy will be helped by the creation of more efficient nanodelivery technologies and extensive clinical research. Given that humans and animals differ greatly from one another, it is important to explore their compatible biocompatibilities, release, accumulation and metabolism. In addition to structural modulation, future research needs to investigate the treatment mechanisms, especially at the molecular level. We believe that nanomedicine based redox modulation therapy will bring new and more effective strategies for cancer therapy with significant breakthroughs in the near future.
Drug | Nanoparticles | Status | Tumor type | Identifier |
---|---|---|---|---|
Doxorubicin | Doxil | Unknown | Lymphoma leukemia | NCT03975205 |
Completed | Ovarian cancer | NCT00846612 | ||
Lipodox | Recruiting | Breast cancer; ovarian cancer | NCT05273944 | |
Completed | Ovarian cancer recurrent | NCT03681548 | ||
Myocet | Completed | Malignant glioma | NCT02861222 | |
Paclitaxel | Lipusu | Unknown | Breast cancer | NCT02142790 |
Abraxane | Terminated | Relapsed or refractory multiple myeloma | NCT02075021 | |
Genexol-PM | Terminated | Hepatocellular carcinoma | NCT03008512 | |
Completed | Bladder cancer; ureter cancer | NCT01426126 | ||
Nanoxel | Unknown | Advanced breast cancer | NCT00915369 | |
Paclitaxel albumin‐stabilized nanoparticle formulation | Withdrawn | Non-muscle Invasive Bladder Cancer (NMIBC) | NCT02718742 | |
NanoPac | Completed | Adenocarcinoma of the prostate | NCT03077659 | |
Radiation; hafnium oxide | NBTXR3 | Completed | Adult soft tissue sarcom | NCT01433068 |
Radiation; ferumoxytol | SPION | Recruiting | Liver neoplasms; hepatic cirrhosis; hepatic carcinoma | NCT04682847 |
References
1 Hayes JD, Dinkova-Kostova AT, Tew KD. Oxidative stress in cancer. Cancer Cell 2020; 38: 167-197.
| Crossref | Google Scholar |
2 Nie Y, Chen W, Kang Y, Yuan X, Li Y, Zhou J, et al. Two-dimensional porous vermiculite-based nanocatalysts for synergetic catalytic therapy. Biomaterials. 2023; 295: 122031.
| Crossref | Google Scholar |
3 Li B, Sun C, Lin X, Busch W. The emerging role of GSNOR in oxidative stress regulation. Trends Plant Sci 2021; 26: 156-168.
| Crossref | Google Scholar |
4 Morry J, Ngamcherdtrakul W, Yantasee W. Oxidative stress in cancer and fibrosis: Opportunity for therapeutic intervention with antioxidant compounds, enzymes, and nanoparticles. Redox Biol 2017; 11: 240-253.
| Crossref | Google Scholar |
5 Jiang J, Peng L, Wang K, Huang C. Moonlighting metabolic enzymes in cancer: New perspectives on the redox code. Antioxid Redox Signal 2021; 34: 979-1003.
| Crossref | Google Scholar |
6 Zuo L, Wijegunawardana D. Redox role of ROS and inflammation in pulmonary diseases. In: Wang YX, editor. Lung inflammation in health and disease, Vol. II. Advances in experimental medicine and biology. Vol. 1304. Cham: Springer; 2021. pp. 187–204. Żukowski P, Maciejczyk M, Waszkiel D. Sources of free radicals and oxidative stress in the oral cavity. Arch Oral Biol 2018; 92: 8-17.
| Crossref | Google Scholar |
7 Zhu Y-p, Zheng Z, Hu S, Ru X, Fan Z, Qiu L, et al. Unification of opposites between two antioxidant transcription factors Nrf1 and Nrf2 in mediating distinct cellular responses to the endoplasmic reticulum stressor tunicamycin. Antioxidants (Basel) 2019; 9: 4.
| Crossref | Google Scholar |
8 Iqbal Z, Sarkhosh A, Balal RM, Gómez C, Zubair M, Ilyas N, et al. Silicon alleviate hypoxia stress by improving enzymatic and non-enzymatic antioxidants and regulating nutrient uptake in muscadine grape (Muscadinia rotundifolia Michx.). Front Plant Sci 2020; 11: 618873.
| Crossref | Google Scholar | Casagrande S, Hau M. Enzymatic antioxidants but not baseline glucocorticoids mediate the reproduction–survival trade-off in a wild bird. Proc Biol Sci 2018; 285: 20182141.
| Crossref | Google Scholar |
9 Xiao W, Loscalzo J. Metabolic responses to reductive stress. Antioxid Redox Signal 2020; 32: 1330-1347.
| Crossref | Google Scholar |
10 Peng L, Jiang J, Chen H-N, Zhou L, Huang Z, Qin S, et al. Redox-sensitive cyclophilin a elicits chemoresistance through realigning cellular oxidative status in colorectal cancer. Cell Rep 2021; 37: 110069.
| Crossref | Google Scholar |
11 Klaunig JE. Oxidative stress and cancer. Curr Pharm Des 2018; 24: 4771-4778.
| Crossref | Google Scholar |
12 Xu J, Zhang S, Wang R, Wu X, Zeng L, Fu Z. Knockdown of PRDX2 sensitizes colon cancer cells to 5-FU by suppressing the PI3K/AKT signaling pathway. Biosci Rep 2017; 37: BSR20160447.
| Crossref | Google Scholar |
13 Sun Y, Berleth N, Wu W, Schlütermann D, Deitersen J, Stuhldreier F, et al. Fin56-induced ferroptosis is supported by autophagy-mediated GPX4 degradation and functions synergistically with mTOR inhibition to kill bladder cancer cells. Cell Death Dis 2021; 12: 1028.
| Crossref | Google Scholar |
14 Azmanova M, Pitto-Barry A. Oxidative stress in cancer therapy: Friend or enemy? Chembiochem. 2022; 23: e202100641.
| Crossref | Google Scholar |
15 Albalawi AE, Alanazi AD, Baharvand P, Sepahvand M, Mahmoudvand H. High Potency of Organic and Inorganic Nanoparticles to Treat Cystic Echinococcosis: An Evidence-Based Review. Nanomaterials (Basel) 2020; 10(12):.
| Crossref | Google Scholar |
16 Liu W, Su J, Shi Q, Wang J, Chen X, Zhang S, Li M, Cui J, Fan C, Sun B, Wang G. RGD Peptide-Conjugated Selenium Nanocomposite Inhibits Human Glioma Growth by Triggering Mitochondrial Dysfunction and ROS-Dependent MAPKs Activation. Front Bioeng Biotechnol 2021; 9: 781608.
| Crossref | Google Scholar |
17 Yang M, Li J, Gu P, Fan X. The application of nanoparticles in cancer immunotherapy: Targeting tumor microenvironment. Bioact Mater 2021; 6: 1973-1987.
| Crossref | Google Scholar | Roma-Rodrigues C, Mendes R, Baptista PV, Fernandes AR. Targeting tumor microenvironment for cancer therapy. Int J Mol Sci 2019; 20: 840.
| Crossref | Google Scholar |
18 Chen M, Liu D, Liu F, Wu Y, Peng X, Song F. Recent advances of redox-responsive nanoplatforms for tumor theranostics. J Control Release 2021; 332: 269-284.
| Crossref | Google Scholar |
19 Tian H, Zhang T, Qin S, Huang Z, Zhou L, Shi J, et al. Enhancing the therapeutic efficacy of nanoparticles for cancer treatment using versatile targeted strategies. J Hematol Oncol 2022; 15(1): 132.
| Crossref | Google Scholar |
20 Li X-Y, Deng F-A, Zheng R-R, Liu L-S, Liu Y-B, Kong R-J, et al. Carrier free photodynamic synergists for oxidative damage amplified tumor therapy. Small. 2021; 17: e2102470.
| Crossref | Google Scholar |
21 Chen T, Zeng W, Tie C, Yu M, Hao H, Deng Y, et al. Engineered gold/black phosphorus nanoplatforms with remodeling tumor microenvironment for sonoactivated catalytic tumor theranostics. Bioact Mater 2022; 10: 515-525.
| Crossref | Google Scholar |
22 Quiles JL, Sánchez-González C, Vera-Ramírez L, Giampieri F, Navarro-Hortal MD, Xiao J, et al. Reductive stress, bioactive compounds, redox-active metals, and dormant tumor cell biology to develop redox-based tools for the treatment of cancer. Antioxid Redox Signal 2020; 33: 860-881.
| Crossref | Google Scholar |
23 Tan G-R, Hsu C-YS, Zhang Y. pH-responsive hybrid nanoparticles for imaging spatiotemporal pH changes in biofilm-dentin microenvironments. ACS Appl Mater Interfaces 2021; 13: 46247-46259.
| Crossref | Google Scholar | Xie N, Shen G, Gao W, Huang Z, Huang C, Fu L. Neoantigens: Promising targets for cancer therapy. Signal Transduct Target Ther 2023; 8: 9.
| Crossref | Google Scholar |
24 Ge L, Qiao C, Tang Y, Zhang X, Jiang X. Light-activated hypoxia-sensitive covalent organic framework for tandem-responsive drug delivery. Nano Lett 2021; 21: 3218-3224.
| Crossref | Google Scholar |
25 Li Y, Jeon J, Park JH. Hypoxia-responsive nanoparticles for tumor-targeted drug delivery. Cancer Lett 2020; 490: 31-43.
| Crossref | Google Scholar | Filipczak N, Joshi U, Attia SA, Berger Fridman I, Cohen S, Konry T, et al. Hypoxia-sensitive drug delivery to tumors. J Control Release 2022; 341: 431-442.
| Crossref | Google Scholar |
26 Gisbert-Garzarán M, Berkmann JC, Giasafaki D, Lozano D, Spyrou K, Manzano M, et al. Engineered pH-responsive mesoporous carbon nanoparticles for drug delivery. ACS Appl Mater Interfaces 2020; 12: 14946-14957.
| Crossref | Google Scholar | Xu Y, Zi Y, Lei J, Mo X, Shao Z, Wu Y, et al. pH-responsive nanoparticles based on cholesterol/imidazole modified oxidized-starch for targeted anticancer drug delivery. Carbohydr Polym 2020; 233: 115858.
| Crossref | Google Scholar |
27 Niu B, Liao K, Zhou Y, Wen T, Quan G, Pan X, et al. Application of glutathione depletion in cancer therapy: Enhanced ROS-based therapy, ferroptosis, and chemotherapy. Biomaterials. 2021; 277: 121110.
| Crossref | Google Scholar |
28 Tang Q, Cheng Z, Yang N, Li Q, Wang P, Chen D, et al. Hydrangea-structured tumor microenvironment responsive degradable nanoplatform for hypoxic tumor multimodal imaging and therapy. Biomaterials. 2019; 205: 1-10.
| Crossref | Google Scholar |
29 Lin LS, Huang T, Song J, Ou XY, Wang Z, Deng H, et al. Synthesis of copper peroxide nanodots for H2O2 self-supplying chemodynamic therapy. J Am Chem Soc 2019; 141: 9937-9945.
| Crossref | Google Scholar |
30 Wang X, Wang X, Jin S, Muhammad N, Guo Z. Stimuli-responsive therapeutic metallodrugs. Chem Rev 2019; 119: 1138-1192.
| Crossref | Google Scholar |
31 Ling X, Tu J, Wang J, Shajii A, Kong N, Feng C, et al. Glutathione-responsive prodrug nanoparticles for effective drug delivery and cancer therapy. ACS Nano 2019; 13: 357-370.
| Crossref | Google Scholar |
32 Gong N, Ma X, Ye X, Zhou Q, Chen X, Tan X, et al. Carbon-dot-supported atomically dispersed gold as a mitochondrial oxidative stress amplifier for cancer treatment. Nat Nanotechnol 2019; 14: 379-387.
| Crossref | Google Scholar |
33 Liu C, Wang D, Zhang S, Cheng Y, Yang F, Xing Y, et al. Biodegradable biomimic copper/manganese silicate nanospheres for chemodynamic/photodynamic synergistic therapy with simultaneous glutathione depletion and hypoxia relief. ACS Nano 2019; 13: 4267-4277.
| Crossref | Google Scholar |
34 Wang Y, Li Y, Zhang Z, Wang L, Wang D, Tang BZ. Triple-jump photodynamic theranostics: MnO2 combined upconversion nanoplatforms involving a type-I photosensitizer with aggregation-induced emission characteristics for potent cancer treatment. Adv Mater 2021; 33: e2103748.
| Crossref | Google Scholar |
35 Fan Y, Li P, Hu B, Liu T, Huang Z, Shan C, et al. A smart photosensitizer-cerium oxide nanoprobe for highly selective and efficient photodynamic therapy. Inorg Chem 2019; 58: 7295-7302.
| Crossref | Google Scholar |
36 Kuang Y, Balakrishnan K, Gandhi V, Peng X. Hydrogen peroxide inducible DNA cross-linking agents: Targeted anticancer prodrugs. J Am Chem Soc 2011; 133: 19278-19281.
| Crossref | Google Scholar |
37 Guo P, Wang K, Jin W-J, Xie H, Qi L, Liu X-Y, et al. Dynamic kinetic cross-electrophile arylation of benzyl alcohols by nickel catalysis. J Am Chem Soc 2021; 143: 513-523.
| Crossref | Google Scholar |
38 Sun S, Chen Q, Tang Z, Liu C, Li Z, Wu A, et al. Tumor microenvironment stimuli-responsive fluorescence imaging and synergistic cancer therapy by carbon-dot–Cu2+ nanoassemblies. Angew Chem Int Ed Engl 2020; 59: 21041-21048.
| Crossref | Google Scholar |
39 Ding H, Cai Y, Gao L, Liang M, Miao B, Wu H, et al. Exosome-like nanozyme vesicles for H2O2-responsive catalytic photoacoustic imaging of xenograft nasopharyngeal carcinoma. Nano Lett 2019; 19: 203-209.
| Crossref | Google Scholar |
40 Zeng Q, Zhang R, Zhang T, Xing D. H2O2-responsive biodegradable nanomedicine for cancer-selective dual-modal imaging guided precise photodynamic therapy. Biomaterials. 2019; 207: 39-48.
| Crossref | Google Scholar |
41 He H, Meng S, Li H, Yang Q, Xu Z, Chen X, et al. Nanoplatform based on GSH-responsive mesoporous silica nanoparticles for cancer therapy and mitochondrial targeted imaging. Mikrochim Acta 2021; 188: 154.
| Crossref | Google Scholar |
42 Xu J, Han W, Cheng Z, Yang P, Bi H, Yang D, et al. Bioresponsive and near infrared photon co-enhanced cancer theranostic based on upconversion nanocapsules. Chem Sci 2018; 9: 3233-3247.
| Crossref | Google Scholar |
43 Bertoni S, Machness A, Tiboni M, Bártolo R, Santos HA. Reactive oxygen species responsive nanoplatforms as smart drug delivery systems for gastrointestinal tract targeting. Biopolymers. 2020; 111: e23336.
| Crossref | Google Scholar |
44 Yang B, Wang K, Zhang D, Sun B, Ji B, Wei L, et al. Light-activatable dual-source ROS-responsive prodrug nanoplatform for synergistic chemo-photodynamic therapy. Biomater Sci 2018; 6: 2965-2975.
| Crossref | Google Scholar |
45 Lee HL, Hwang SC, Nah JW, Kim J, Cha B, Kang DH, et al. Redox- and pH-responsive nanoparticles release piperlongumine in a stimuli-sensitive manner to inhibit pulmonary metastasis of colorectal carcinoma cells. J Pharm Sci 2018; 107: 2702-2712.
| Crossref | Google Scholar |
46 Xie A, Hanif S, Ouyang J, Tang Z, Kong N, Kim NY, et al. Stimuli-responsive prodrug-based cancer nanomedicine. EBioMedicine. 2020; 56: 102821.
| Crossref | Google Scholar |
47 He L, Sun M, Cheng X, Xu Y, Lv X, Wang X, et al. pH/redox dual-sensitive platinum (IV)-based micelles with greatly enhanced antitumor effect for combination chemotherapy. J Colloid Interface Sci 2019; 541: 30-41.
| Crossref | Google Scholar |
48 Han L, Zhang X-Y, Wang Y-L, Li X, Yang X-H, Huang M, et al. Redox-responsive theranostic nanoplatforms based on inorganic nanomaterials. J Control Release 2017; 259: 40-52.
| Crossref | Google Scholar | Yang J, Pan S, Gao S, Dai Y, Xu H. Anti-recurrence/metastasis and chemosensitization therapy with thioredoxin reductase-interfering drug delivery system. Biomaterials 2020; 249: 120054.
| Crossref | Google Scholar |
49 Wang Y, Shahi PK, Wang X, Xie R, Zhao Y, Wu M, et al. In vivo targeted delivery of nucleic acids and CRISPR genome editors enabled by GSH-responsive silica nanoparticles. J Control Release 2021; 336: 296-309.
| Crossref | Google Scholar |
50 Li L, Tian H, Zhang Z, Ding N, He K, Lu S, et al. Carrier-free nanoplatform via evoking pyroptosis and immune response against breast cancer. ACS Appl Mater Interfaces 2023; 15: 452-468.
| Crossref | Google Scholar |
51 Yang Z, Li P, Chen Y, Gan Q, Feng Z, Jin Y, et al. Construction of pH/glutathione responsive chitosan nanoparticles by a self-assembly/self-crosslinking method for photodynamic therapy. Int J Biol Macromol 2021; 167: 46-58.
| Crossref | Google Scholar | El-Shorbagy HM, Eissa SM, Sabet S, El-Ghor AA. Apoptosis and oxidative stress as relevant mechanisms of antitumor activity and genotoxicity of ZnO-NPs alone and in combination with N-acetyl cysteine in tumor-bearing mice. Int J Nanomedicine 2019; 14: 3911-3928.
| Crossref | Google Scholar |
52 Yao Q, Chen R, Ganapathy V, Kou L. Therapeutic application and construction of bilirubin incorporated nanoparticles. J Control Release 2020; 328: 407-424.
| Crossref | Google Scholar |
53 Afzal O, Akhter MH, Ahmad I, Muzammil K, Dawria A, Zeyaullah M, et al. A β-sitosterol encapsulated biocompatible alginate/chitosan polymer nanocomposite for the treatment of breast cancer. Pharmaceutics. 2022; 14: 1711.
| Crossref | Google Scholar |
54 Zhu X, Lei X, Wang J, Dong W. Protective effects of resveratrol on hyperoxia-induced lung injury in neonatal rats by alleviating apoptosis and ROS production. J Matern Fetal Neonatal Med 2020; 33: 4150-4158.
| Crossref | Google Scholar | Yan B, Cheng L, Jiang Z, Chen K, Zhou C, Sun L, et al. Resveratrol inhibits ROS-promoted activation and glycolysis of pancreatic stellate cells via suppression of miR-21. Oxid Med Cell Longev 2018; 2018: 1346958.
| Crossref | Google Scholar |
55 Shi Q, Wang X, Tang X, Zhen N, Wang Y, Luo Z, et al. In vitro antioxidant and antitumor study of zein/SHA nanoparticles loaded with resveratrol. Food Sci Nutr 2021; 9: 3530-3537.
| Crossref | Google Scholar |
56 Wu T, Liu Y, Cao Y, Liu Z. Engineering macrophage exosome disguised biodegradable nanoplatform for enhanced sonodynamic therapy of glioblastoma. Adv Mater 2022; 34: e2110364.
| Crossref | Google Scholar |
57 Ding B, Zheng P, Ma P, Lin J. Manganese oxide nanomaterials: Synthesis, properties, and theranostic applications. Adv Mater 2020; 32: e1905823.
| Crossref | Google Scholar |
58 Zhang R, Song X, Liang C, Yi X, Song G, Chao Y, et al. Catalase-loaded cisplatin-prodrug-constructed liposomes to overcome tumor hypoxia for enhanced chemo-radiotherapy of cancer. Biomaterials. 2017; 138: 13-21.
| Crossref | Google Scholar |
59 Zeng Z, He X, Li C, Lin S, Chen H, Liu L, et al. Oral delivery of antioxidant enzymes for effective treatment of inflammatory disease. Biomaterials. 2021; 271: 120753.
| Crossref | Google Scholar |
60 Jiso A, Demuth P, Bachowsky M, Haas M, Seiwert N, Heylmann D, et al. Natural merosesquiterpenes activate the DNA damage response via DNA strand break formation and trigger apoptotic cell death in p53-wild-type and mutant colorectal cancer. Cancers (Basel) 2021; 13: 3282.
| Crossref | Google Scholar | Lim W, Park S, Bazer FW, Song G. Naringenin-induced apoptotic cell death in prostate cancer cells is mediated via the PI3K/AKT and MAPK signaling pathways. J Cell Biochem 2017; 118: 1118-1131.
| Crossref | Google Scholar | Xie Q, Chen Y, Tan H, Liu B, Zheng L-L, Mu Y. Targeting autophagy with natural compounds in cancer: A renewed perspective from molecular mechanisms to targeted therapy. Front Pharmacol 2021; 12: 748149.
| Crossref | Google Scholar |
61 Horie M, Tabei Y. Role of oxidative stress in nanoparticles toxicity. Free Radic Res 2021; 55: 331-342.
| Crossref | Google Scholar |
62 Ming H, Li B, Tian H, Zhou L, Jiang J, Zhang T, et al. A minimalist and robust chemo-photothermal nanoplatform capable of augmenting autophagy-modulated immune response against breast cancer. Mater Today Bio 2022; 15: 100289.
| Crossref | Google Scholar |
63 Lu S, Tian H, Li L, Li B, Yang M, Zhou L, et al. Nanoengineering a zeolitic imidazolate framework-8 capable of manipulating energy metabolism against cancer chemo-phototherapy resistance. Small. 2022; 18: e2204926.
| Crossref | Google Scholar |
64 Jia W, Tian H, Jiang J, Zhou L, Li L, Luo M, et al. Brain-targeted HFn-Cu-REGO nanoplatform for site-specific delivery and manipulation of autophagy and cuproptosis in glioblastoma. Small. 2023; 19: e2205354.
| Crossref | Google Scholar |
65 Lin L-S, Wang J-F, Song J, Liu Y, Zhu G, Dai Y, Shen Z, et al. Cooperation of endogenous and exogenous reactive oxygen species induced by zinc peroxide nanoparticles to enhance oxidative stress-based cancer therapy. Theranostics 2019; 9(24): 7200-7209.
| Crossref | Google Scholar |
66 El Yamani N, Collins AR, Rundén-Pran E, Fjellsbø LM, Shaposhnikov S, Zienolddiny S, et al. In vitro genotoxicity testing of four reference metal nanomaterials, titanium dioxide, zinc oxide, cerium oxide and silver: Towards reliable hazard assessment. Mutagenesis. 2017; 32: 117-126.
| Crossref | Google Scholar |
67 Makumire S, Chakravadhanula VSK, Köllisch G, Redel E, Shonhai A. Immunomodulatory activity of zinc peroxide (ZnO2) and titanium dioxide (TiO2) nanoparticles and their effects on DNA and protein integrity. Toxicol Lett 2014; 227: 56-64.
| Crossref | Google Scholar |
68 Karimi M, Sahandi Zangabad P, Baghaee-Ravari S, Ghazadeh M, Mirshekari H, Hamblin MR. Smart nanostructures for cargo delivery: Uncaging and activating by light. J Am Chem Soc 2017; 139: 4584-4610.
| Crossref | Google Scholar | Zhang L, Wang D, Yang K, Sheng D, Tan B, Wang Z, et al. Mitochondria-targeted artificial “nano-RBCs” for amplified synergistic cancer phototherapy by a single NIR irradiation. Adv Sci (Weinh) 2018; 5: 1800049.
| Crossref | Google Scholar | Jin P, Jiang J, Zhou L, Huang Z, Nice EC, Huang C, et al. Mitochondrial adaptation in cancer drug resistance: Prevalence, mechanisms, and management. J Hematol Oncol 2022; 15: 97.
| Crossref | Google Scholar |
69 Yang N, Xiao W, Song X, Wang W, Dong X. Recent advances in tumor microenvironment hydrogen peroxide-responsive materials for cancer photodynamic therapy. Nano-Micro Lett 2020; 12: 15.
| Crossref | Google Scholar |
70 Machuca A, Garcia-Calvo E, Anunciação DS, Luque-Garcia JL. Rhodium Nanoparticles as a Novel Photosensitizing Agent in Photodynamic Therapy against Cancer. Chemistry 2020; 26(34): 7685-7691.
| Crossref | Google Scholar |
71 Dai J, Song J, Qiu Y, Wei J, Hong Z, Li L, et al. Gold nanoparticle-decorated g-C3N4 nanosheets for controlled generation of reactive oxygen species upon 670 nm laser illumination. ACS Appl Mater Interfaces 2019; 11: 10589-10596.
| Crossref | Google Scholar |
72 Rastinehad AR, Anastos H, Wajswol E, Winoker JS, Sfakianos JP, Doppalapudi SK, et al. Gold nanoshell-localized photothermal ablation of prostate tumors in a clinical pilot device study. Proc Natl Acad Sci U S A 2019; 116: 18590-18596.
| Crossref | Google Scholar |
73 Baskaran R, Lee J, Yang S-G. Clinical development of photodynamic agents and therapeutic applications. Biomater Res 2018; 22: 25.
| Crossref | Google Scholar |
74 Pan Y, Zhu Y, Xu C, Pan C, Shi Y, Zou J, et al. Biomimetic yolk-shell nanocatalysts for activatable dual-modal-image-guided triple-augmented chemodynamic therapy of cancer. ACS Nano 2022; 16: 19038-19052.
| Crossref | Google Scholar |
75 Zhang W, Liu C, Liu Z, Zhao C, Zhu J, Ren J, et al. A cell selective fluoride-activated MOF biomimetic platform for prodrug synthesis and enhanced synergistic cancer therapy. ACS Nano 2022; 16: 20975-20984.
| Crossref | Google Scholar | Li X, Zhou Q, Japir AA-WMM, Dutta D, Lu N, Ge Z. Protein-delivering nanocomplexes with fenton reaction-triggered cargo release to boost cancer immunotherapy. ACS Nano 2022; 16: 14982-14999.
| Crossref | Google Scholar |
76 Zhang L, Li C-X, Wan S-S, Zhang X-Z. Nanocatalyst-mediated chemodynamic tumor therapy. Adv Healthc Mater 2022; 11: e2101971.
| Crossref | Google Scholar |
77 Lin K, Lin Z, Li Y, Zheng Y, Zhang D. Ultrasound-induced reactive oxygen species generation and mitochondria-specific damage by sonodynamic agent/metal ion-doped mesoporous silica. RSC Adv 2019; 9: 39924-39931.
| Crossref | Google Scholar | Guo X, Yang N, Ji W, Zhang H, Dong X, Zhou Z, et al. Mito-bomb: Targeting mitochondria for cancer therapy. Adv Mater 2021; 33: e2007778.
| Crossref | Google Scholar |
78 Huang J, Xiao Z, An Y, Han S, Wu W, Wang Y, et al. Nanodrug with dual-sensitivity to tumor microenvironment for immuno-sonodynamic anti-cancer therapy. Biomaterials. 2021; 269: 120636.
| Crossref | Google Scholar |
79 Shakeri A, Cicero AFG, Panahi Y, Mohajeri M, Sahebkar A. Curcumin: A naturally occurring autophagy modulator. J Cell Physiol 2019; 234: 5643-5654.
| Crossref | Google Scholar | Tavana E, Mollazadeh H, Mohtashami E, Modaresi SMS, Hosseini A, Sabri H, et al. Quercetin: A promising phytochemical for the treatment of glioblastoma multiforme. Biofactors 2020; 46: 356-366.
| Crossref | Google Scholar |
80 Kundu M, Sadhukhan P, Ghosh N, Chatterjee S, Manna P, Das J, et al. pH-responsive and targeted delivery of curcumin via phenylboronic acid-functionalized ZnO nanoparticles for breast cancer therapy. J Adv Res 2019; 18: 161-172.
| Crossref | Google Scholar |
81 Sadhukhan P, Kundu M, Chatterjee S, Ghosh N, Manna P, Das J, et al. Targeted delivery of quercetin via pH-responsive zinc oxide nanoparticles for breast cancer therapy. Mater Sci Eng C Mater Biol Appl 2019; 100: 129-140.
| Crossref | Google Scholar |
82 Yang N, Zheng R-R, Chen Z-Y, Wang R-X, Zhao L-P, Chen X-Y, et al. A carrier free photodynamic oxidizer for enhanced tumor therapy by redox homeostasis disruption. Biomater Sci 2022; 10: 1575-1581.
| Crossref | Google Scholar |
83 Li B, Jiang J, Assaraf YG, Xiao H, Chen Z-S, Huang C. Surmounting cancer drug resistance: New insights from the perspective of N6-methyladenosine RNA modification. Drug Resist Updat 2020; 53: 100720.
| Crossref | Google Scholar | Jin P, Jiang J, Zhou L, Huang Z, Qin S, Chen H-N, et al. Disrupting metformin adaptation of liver cancer cells by targeting the TOMM34/ATP5B axis. EMBO Mol Med 2022; 14: e16082.
| Crossref | Google Scholar |
84 Shi J, Kantoff PW, Wooster R, Farokhzad OC. Cancer nanomedicine: Progress, challenges and opportunities. Nat Rev Cancer 2017; 17: 20-37.
| Crossref | Google Scholar |
85 Lin L-S, Wang J-F, Song J, Liu Y, Zhu G, Dai Y, et al. Cooperation of endogenous and exogenous reactive oxygen species induced by zinc peroxide nanoparticles to enhance oxidative stress-based cancer therapy. Theranostics. 2019; 9: 7200-7209.
| Crossref | Google Scholar |