Climate change effects on pasture systems in south-eastern Australia
B. R. Cullen A G , I. R. Johnson B , R. J. Eckard A , G. M. Lodge C , R. G. Walker D , R. P. Rawnsley E and M. R. McCaskill FA Melbourne School of Land and Environment, University of Melbourne, Vic. 3010, Australia.
B IMJ Consultants, Armidale, NSW 2350, Australia.
C NSW Department of Primary Industries, Tamworth Agricultural Institute, NSW 2340, Australia.
D Queensland Primary Industries and Fisheries, Mutdapilly Research Station, Peak Crossing, Qld 4306, Australia.
E Tasmanian Institute of Agricultural Research, University of Tasmania, Burnie, Tas. 7320, Australia.
F Victorian Department of Primary Industries, Hamilton, Vic. 3300, Australia.
G Corresponding author. Email: bcullen@unimelb.edu.au
Crop and Pasture Science 60(10) 933-942 https://doi.org/10.1071/CP09019
Submitted: 16 January 2009 Accepted: 13 July 2009 Published: 18 September 2009
Abstract
Climate change projections for Australia predict increasing temperatures, changes to rainfall patterns, and elevated atmospheric carbon dioxide (CO2) concentrations. The aims of this study were to predict plant production responses to elevated CO2 concentrations using the SGS Pasture Model and DairyMod, and then to quantify the effects of climate change scenarios for 2030 and 2070 on predicted pasture growth, species composition, and soil moisture conditions of 5 existing pasture systems in climates ranging from cool temperate to subtropical, relative to a historical baseline. Three future climate scenarios were created for each site by adjusting historical climate data according to temperature and rainfall change projections for 2030, 2070 mid- and 2070 high-emission scenarios, using output from the CSIRO Mark 3 global climate model. In the absence of other climate changes, mean annual pasture production at an elevated CO2 concentration of 550 ppm was predicted to be 24–29% higher than at 380 ppm CO2 in temperate (C3) species-dominant pastures in southern Australia, with lower mean responses in a mixed C3/C4 pasture at Barraba in northern New South Wales (17%) and in a C4 pasture at Mutdapilly in south-eastern Queensland (9%). In the future climate scenarios at the Barraba and Mutdapilly sites in subtropical and subhumid climates, respectively, where climate projections indicated warming of up to 4.4°C, with little change in annual rainfall, modelling predicted increased pasture production and a shift towards C4 species dominance. In Mediterranean, temperate, and cool temperate climates, climate change projections indicated warming of up to 3.3°C, with annual rainfall reduced by up to 28%. Under future climate scenarios at Wagga Wagga, NSW, and Ellinbank, Victoria, our study predicted increased winter and early spring pasture growth rates, but this was counteracted by a predicted shorter spring growing season, with annual pasture production higher than the baseline under the 2030 climate scenario, but reduced by up to 19% under the 2070 high scenario. In a cool temperate environment at Elliott, Tasmania, annual production was higher than the baseline in all 3 future climate scenarios, but highest in the 2070 mid scenario. At the Wagga Wagga, Ellinbank, and Elliott sites the effect of rainfall declines on pasture production was moderated by a predicted reduction in drainage below the root zone and, at Ellinbank, the use of deeper rooted plant systems was shown to be an effective adaptation to mitigate some of the effect of lower rainfall.
Additional keywords: CO2 concentration, C4 species, C3 species, pasture production, water balance.
Introduction
Climate change projections for Australia indicate increasing temperatures, changes to rainfall patterns, and elevated atmospheric carbon dioxide (CO2) concentrations (CSIRO and BoM 2007), all of which are likely to affect the productivity of pasture-based systems. The overall effect of future climate changes on pasture production is uncertain and likely to vary regionally, depending on the combination of changes to temperature and rainfall, as well as plant responses to elevated atmospheric CO2 concentrations (Harle et al. 2007; Howden et al. 2008; McKeon et al. 2009). Temperature projections for Australia predict warming of 0.4–1.8°C by 2030 and 2.2–5°C by 2070 under high-emission scenarios (A1FI storyline, IPCC 2000), with larger increases in inland compared with southern Australia (CSIRO and BoM 2007). There is considerable variation in projected rainfall patterns. The annual rainfall projections for 2030 range from –10 to +5% across northern Australia and from –10% to no change in southern Australia, while under 2070 high-emission scenarios (A1FI), projected changes are for –30 to +20% annual rainfall in northern, central, and eastern Australia, and –30 to +5% annual rainfall across southern Australia (CSIRO and BoM 2007). In southern Australia the rainfall reductions are projected to be largest in winter and spring.
In addition to changes in temperature and soil moisture, plant production will be influenced by elevated atmospheric CO2 concentrations through increased photosynthetic and water-use efficiencies (e.g. Ainsworth and Long 2005), resulting in increased biomass production (Long et al. 2004; Lüsher et al. 2006). The magnitude of the plant production response to elevated CO2 concentrations will be determined by interactions among pasture type, soil moisture, and soil nutrient availability (Stokes and Ash 2007). Across the Australian rangelands, McKeon et al. (2009) demonstrated that elevated CO2 levels could enhance the positive effect of higher rainfall future climate scenarios on forage production and mitigate the effect of reduced production in lower rainfall scenarios. Howden et al. (2008) suggested that increased production from elevated CO2 would be offset by a 10% rainfall reduction; however, there is a need to evaluate the potential effects of future climate scenarios on Australian grazing systems across a range of regions and pasture types (Harle et al. 2007). Biophysical modelling approaches which integrate climatic changes with plant responses to elevated CO2 concentrations, such as those used in cropping systems (e.g. van Ittersum et al. 2003; Anwar et al. 2007), are the only means available to do this.
Climate change may also affect grazing systems by altering species composition; for example, warming will favour tropical (C4) species over temperate (C3) species (Howden et al. 2008). In most cases, C3 species are of higher forage quality than C4 species, and are expected to remain so under elevated atmospheric CO2 conditions (Barbehenn et al. 2004), so the effect of climatic change on the balance between C3 and C4 species could have important implications for animal production. In addition, changes to rainfall patterns may influence natural resource degradation processes such as erosion and salinity through changes in runoff and drainage patterns (van Ittersum et al. 2003; Howden et al. 2008).
The objective of this study was to quantify the net effect of future climate scenarios on pasture production systems, using the biophysical grazing systems models DairyMod and the SGS Pasture Model (Johnson et al. 2003, 2008). The specific aims were: (1) to predict plant production responses to elevated CO2 concentrations; (2) to quantify the net effect of 3 future climate scenarios, based on regional projections for changes to rainfall, temperature, solar radiation, relative humidity, and atmospheric CO2 concentrations in 2030 and 2070, on the production, species composition, and soil water balance of 5 current pasture systems in eastern Australia; and (3) where large reductions in pasture dry matter (DM) production were predicted, to examine if these changes could be mitigated by increasing plant root depth. The pasture systems simulated covered a range of climates from subtropical to cool temperate and pastures that were dominated by C4 and C3 species.
Materials and methods
Sites and pasture systems simulations
The effects of future climate scenarios on pastoral systems were modelled at 5 sites in eastern Australia, ranging from a C4-dominant pasture in subtropical south-eastern Queensland to a C3 pasture in the cool temperate environment of north-western Tasmania. Site details, including location, climatic zone, and pasture species, are shown in Table 1. All sites were in the medium–high rainfall zone, with the Wagga Wagga site having the lowest mean annual rainfall (565 mm). The SGS Pasture Model and DairyMod version 4.7.5 (Johnson et al. 2003, 2008) were used to simulate rainfed (non-irrigated) pasture systems using daily climate data for each site. These models use the same equations for their soil and pasture growth components, and have previously been shown to adequately simulate pasture systems at these and other sites (Cullen et al. 2008; Lodge and Johnson 2008). The climate scenarios developed for each site were modelled without nutrient limitation and using a ‘put and take’ grazing system, whereby animal numbers were adjusted daily to maintain pasture mass at 2 tonnes (t) DM/ha and stock were removed when it was less than this value. This system was applied to avoid the use of inappropriate stocking rates across the climate scenarios, which may have otherwise biased the model predictions. The effect of climate scenarios on monthly and annual pasture production, species composition, and the water balance (annual runoff and drainage) at each site was investigated.
![]() |
Climate scenarios
An historical baseline climate and 3 future climate scenarios were used to compare the effects of climate change on each of the pasture systems. A 30-year climate ‘baseline’ (1971–2000) was used to represent inherent climate variability at each site. Although the period 1961–90 is often used as the baseline for climate change effect analysis, 1971–2000 was adopted as the baseline in this study since it may better reflect recent climate, following the convention of Hennessy (2007). Three future climate scenarios were developed for each site by adjusting baseline climate data with climate change projections for 2030 and 2070, to create 30-year realisations of each future climate scenario. A 10-year lead-in period, based on 1961–70 historical climate data, was modelled before each scenario to stabilise the initial conditions of the model, but these data were excluded from the analysis.
For each site, historical daily climate data for the 30-year baseline and 10-year lead-in period (i.e. 1 January 1961–31 December 2000) were obtained in April 2008 from the SILO database (www.longpaddock.qld.gov.au/silo/, Jeffrey et al. 2001). Patch-point climate datasets were used for Ellinbank, Elliott, and Mutdapilly (Amberley, 15 km north of the site), while interpolated climate data were used at Barraba and Wagga Wagga. Monthly average minimum and maximum temperature and rainfall over the 30-year baseline period were tested for the presence of any linear annual trends (Anwar et al. 2007). Few significant trends were observed (as measured by r2 correlation) and so de-trending of the data was not undertaken. The lack of significant trends may have been related to the relatively short time-frame (i.e. 30 years) for the dataset examined.
The 3 future climate scenarios created for effect analysis were based on a high greenhouse gas emission scenario in 2030, and mid- and high-emission scenarios in 2070 (hereafter referred to as the ‘2030’, ‘2070 mid’, and ‘2070 high’ future climate scenarios). The mid climate change effect scenario was based on the Intergovernmental Panel on Climate Change (IPCC 2000) A1B emission scenario with medium climate sensitivity, while the 2030 and 2070 high scenarios were based on the IPCC (2000) A1FI emission scenario with high climate sensitivity. For each site and future climate scenario, monthly projections for mean temperature (°C) and rainfall (%) change were obtained from the CSIRO Mark 3 global circulation model, via the OzClim database (www.csiro.au/ozclim). The 3 future climate scenarios were selected to represent a range of climate change effects, i.e. temperature increases of 0.7–1.2°C in the 2030, 1.5–2.6°C in the 2070 mid, and 2.5–4.4°C in the 2070 high scenarios, with associated rainfall changes (Table 2). Climate change projections differed for each site, with larger temperature increases and little change in annual rainfall at the northern sites, compared with smaller temperature increases and annual rainfall declines of up to 28% at the southern sites (Table 2). Monthly, rather than annual, temperature and rainfall change projections from the OzClim database were used so that changes to the seasonal pattern could be included in the future scenarios. There was some variability in month-to-month temperature and rainfall projections from the CSIRO Mark 3 model; however, the climatic changes were consistent with the broad projections for Australia, including the seasonal patterns and regional differences (CSIRO and BoM 2007). For example, the changes suggested reduced spring rainfall and increased summer–autumn rainfall at the northern sites, while in southern Australia the majority of the annual rainfall reduction was projected to occur in winter and spring (Fig. 1). Therefore, these temperature and rainfall change scenarios were within the uncertainty bounds of a range of climate models for these sites and were considered appropriate for assessing climate change effect.
![]() |
![]() |
Daily historical baseline climate data were scaled according to the climate change projections for each future climate scenario, adapting an approach applied in previous climate change effect analyses (e.g. van Ittersum et al. 2003; Anwar et al. 2007). To convert rainfall from the historical baseline to scenario rainfall, daily rainfall was multiplied by a factor from OzClim representing the decrease or increase in rainfall for the relevant month and location. For example, to represent a 15% decrease in rainfall, an historical rainfall of 0.2 mm/day was multiplied by 0.85, giving 0.17 mm for the equivalent scenario day. In preliminary analyses, the number of rain days was reduced and larger rainfall amounts increased to represent greater variability of rainfall, but this made little difference to annual pasture growth (unpublished data).
Daily maximum or minimum temperature (Ťmax or Ťmin) for each scenario was calculated from the historical Tmax or Tmin as:
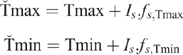
where Is is the increase in mean temperature from OzClim for the relevant month and location, and fs,Tmax and fs,Tmin are scalars to represent the relevant change in maximum or minimum temperature. These scalars were read from maps (CSIRO and BoM 2007, fig. 5.10, p. 60) and were in the ranges 1.0–1.15 for maximum temperature and 0.85–1.0 for minimum temperature, indicating that maximum temperature increased more than the minimum temperature. Finally, annual change statistics for radiation (0.7–2.6% increase) and relative humidity (0.6–2.3% decrease) were incorporated for each scenario by applying scalar factors for the changes from the nearest centre reported by CSIRO and BoM (2007, pp. 130–136). Mean monthly rainfall and minimum and maximum temperatures for each site and future climate scenario are presented in Fig. 1.
The baseline scenario used the current ambient atmospheric CO2 concentration of 380 ppm. This was increased in the 2030 future climate scenario to 455 ppm CO2, and in the 2070 mid and high scenarios to 581 and 716 ppm CO2, respectively (IPCC 2000).
Plant responses to elevated CO2 without other climate changes
Prior to modelling the effects of the 3 future climate scenarios on pasture systems, the annual DM production response to elevated CO2 concentration was modelled at each site by comparing production at 380 ppm CO2 (current ambient) with 550 ppm CO2 in the absence of other changes to climate inputs, using weather data for the baseline scenario (1971–2000). The purpose of these simulations was to demonstrate general agreement between model-predicted DM production responses and measured responses from Free Air CO2 Enhancement (FACE) studies (Long et al. 2004; Ainsworth and Long 2005; Lüsher et al. 2006).
The approach used to incorporate plant responses to elevated atmospheric CO2 concentrations in the SGS Pasture Model and DairyMod is outlined in Appendix 1. In the current study, model responses for leaf photosynthetic potential, plant N content, and canopy conductance were parameterised at 550 ppm CO2 in accordance with experimental results from FACE studies (Table 3). While some care must be taken in directly comparing model responses with experimental results, because the responses measured are relative to different baseline and elevated CO2 concentrations, the comparative values in Table 3 demonstrate that the CO2 effects we used were within the published measured ranges.
Modelling plant adaptation strategies
The effect of increasing the root depth of perennial ryegrass on predicted pasture production under the 2070 high future climate scenario was modelled only at the Ellinbank site. The original root distribution with a maximum root depth of 0.40 m and 50% of roots in the top 0.10 m was compared with a deeper root system, with a maximum root depth of 0.60 m and 50% of roots in the top 0.20 m. Changing root depth had a minimal effect on mean annual root biomass (predicted to be 0.48 and 0.50 t DM/ha, respectively, for the two different root distributions). No other plant characteristics were altered.
Statistical analysis
No formal statistical analysis was applied to the modelled pasture growth and water balance outputs because of inter-correlations in the input climate data and the mechanistic, non-stochastic nature of the models. Trends are presented across the range of future climate scenarios, with the inter-annual variability presented where appropriate.
Results
Predicted production responses to elevated CO2
An elevated atmospheric CO2 concentration of 550 ppm in the absence of other climate changes was associated with mean annual pasture production increases of 24–29% on the C3-dominant pastures at Wagga Wagga, Elliott, and Ellinbank (Table 4). The Mutdapilly pasture had the lowest response (9%), while the mixed C3/C4 pasture response at Barraba was intermediate. The range of responses among years was highest at the Mutdapilly and Barraba sites (Table 4).
Climate change effects
Effects of the 2030, 2070 mid, and 2070 high future climate scenarios on predicted pasture production compared with the baseline climate are shown for all sites in Fig. 2. At the Mutdapilly and Barraba sites, the predicted mean annual DM production increased progressively with each future climate scenario, with a tendency for lower simulated spring–early summer growth, but higher pasture growth rates in the other seasons (Fig. 2a, b). At Mutdapilly, annual average predicted production increased by 3, 10, and 18% over the historical baseline (15.4 t DM/ha) for the 2030, 2070 mid, and 2070 high climate scenarios, respectively. At Barraba the predicted production increases were 16, 41, and 52%, respectively, for the same scenarios over the historical baseline (4 t DM/ha). In the mixed C3/C4 pasture at Barraba, there was also a marked change in the seasonal growth pattern of each species, with a contracted C3 species growing season offset by an increased growing season length and a higher summer growth rate for C4 species (Fig. 3). There was little effect of the future climate scenarios on annual surface runoff at Mutdapilly, where mean runoff was 66–70 mm for all climate scenarios, and a small increase from 69 mm in the baseline to 90 mm in the 2070 high scenario at Barraba. At Mutdapilly there was no simulated drainage below the root zone, while for Barraba the mean annual drainage was <5 mm for all climate scenarios (data not shown).
![]() |
![]() |
At the Wagga Wagga site, mean predicted annual pasture production was slightly higher than the baseline (7.0 t DM/ha) under the 2030 and 2070 mid scenarios (5 and 1%, respectively), but declined by 18% under the 2070 high scenario (Fig. 2c). The simulated future climate scenarios at Wagga Wagga indicated that higher pasture growth rates in winter and early spring were expected with a progressive shortening of the spring growing season (Fig. 2c). A similar change to the seasonal pasture growth pattern was observed in the temperate environment at Ellinbank, with a 4% increase in mean annual pasture DM production in the 2030 scenario compared with the baseline (12.9 t DM/ha), but declines of 3 and 19% under the 2070 mid and 2070 high scenarios, respectively (Fig. 2d). At Elliott, predicted annual pasture production was higher than the baseline (12.5 t DM/ha) under all 3 future climate scenarios, but the increase was highest under the 2070 mid scenario (15%), compared with 10% in the 2030 and 14% in the 2070 high scenarios (Fig. 2e). At all of the southern sites, no predicted runoff occurred for the simulations (data not shown) and drainage was reduced under the future climate scenarios (Fig. 4).
![]() |
Adaptation strategy
The adaptation strategy of increasing root depth from 0.40 to 0.60 m at Ellinbank in the 2070 high climate scenario increased predicted length of the spring growing season and the peak spring growth rate by 10 kg DM/ha (Fig. 5). Mean predicted total annual pasture production increased from 10.5 to 11.6 t DM/ha, but was less than the baseline simulation (12.9 t DM/ha). With the deeper root system, the predicted mean annual drainage was reduced from 270 to 252 mm.
![]() |
Discussion
The expected effect of future climate scenarios on pasture production of 5 systems across south-eastern Australia was determined by modelling how existing well adapted pasture species at each site responded to projected increases in temperature, changes in rainfall patterns, and elevated atmospheric CO2 concentrations. This is the first time that such a comprehensive analysis has been undertaken for a range of sites and pasture types in this region.
Predicted production responses to elevated CO2
The magnitude of the predicted DM production response simulated by raising the atmospheric CO2 concentration from 380 to 550 ppm was dependent on site and pasture system, with the largest increases (22–37%) generally occurring in the C3-dominant pastures in southern Australia and the smallest responses in the C4 pasture at Mutdapilly, in south-eastern Queensland (0–15%, Table 4). These modelled production increases are consistent with comparable results from FACE experiments, particularly where soil nutrients were non-limiting. For example, Lüsher et al. (2006) measured a 7–32% increase in annual DM production at 600 ppm CO2 in an nitrogen (N) fertilised, mixed perennial ryegrass/white clover pasture in Switzerland, with the higher responses recorded at higher levels of soil fertility. Similarly, Long et al. (2004) reported DM increases of 17–22% and –2 to 12% for C3 and C4 species, respectively. Comparable results were also reported by Ainsworth and Long (2005). In our study, the production increases simulated at 550 ppm CO2 were higher than the biomass multipliers used in crop models such as DSSAT-CERES and EPIC/CropSyst [i.e. 11–19% for C3 species and 4–8% for C4 species (Tubiello et al. 2007)], but the magnitude of the difference between responses for C3 and C4 species was similar. The CO2 responses modelled in our study were under non-limiting soil nutrient conditions; however, lower responses would be expected when other factors such as soil N availability are limiting (Lüsher et al. 2006; Newton et al. 2006).
Responses to climate change scenarios
The net effect of future climate scenarios on predicted pasture production was determined by increased plant production under elevated CO2 concentrations, together with responses to higher temperatures and changed rainfall patterns. Howden et al. (2008) suggested that increased plant production from higher CO2 concentrations would be counteracted by a rainfall reduction of ~10%. Our study confirmed this finding for the Mediterranean and temperate climates at Wagga Wagga and Ellinbank, since there was either little change or small increases in simulated annual pasture production when rainfall decreased by up to 10% (i.e. the 2030 and 2070 mid scenarios), but reduced pasture production with larger rainfall reductions (i.e. the 2070 high scenario). However, the modelling for Elliott suggested that this high rainfall, cool temperate environment could cope with larger rainfall declines (>20%) before annual pasture production was reduced. At each of these sites, a change to the seasonal growth pattern was modelled with higher simulated winter and early spring growth rates, reflecting warmer winter temperatures with adequate soil moisture, but the spring growing season was contracted (particularly at Wagga Wagga and Ellinbank, Fig. 2) by higher temperatures and reduced spring rainfall (Fig. 1).
In the subtropical and subhumid climates, increased predicted annual production at the Mutdapilly and Barraba sites appeared to be associated with the heat tolerance of C4 grasses and the small changes in annual rainfall that occurred for each of the climate scenarios (Fig. 1). At both sites, the C4 growing season was extended under the warmer climate scenarios, with a reduction in the C3 growing season at Barraba (Fig. 3), leading to a substantial change in species composition. Since C3 species are expected to have higher forage quality than C4 species at elevated CO2 concentrations, despite some changes in protein and carbohydrate levels (Barbehenn et al. 2004), this shift towards C4 dominance could reduce forage quality, lowering ruminant animal production and increasing methane emissions (Howden et al. 2008).
The main predicted effects of future climate scenarios on the water balance were at the southern Australian sites, with a reduction in drainage below the root zone occurring in the drier climate scenarios (Fig. 4). Similar reductions in drainage were modelled under future climate scenarios in wheat cropping systems in Western Australia (van Ittersum et al. 2003). With less water entering the watertable there are implications for dryland salinity, nitrate leaching, and streamflow. At the Mutdapilly and Barraba sites, there was little change in predicted surface runoff, but projected increases in rainfall intensity (CSIRO and BoM 2007; Alexander and Arblaster 2009) were not taken into account in these climate scenarios. Higher rainfall intensity events may increase runoff and erosion risk, particularly if they occur in combination with longer dry seasons and lower levels of ground cover (Howden et al. 2008).
In dryland pasture systems, deeper rooted perennial plants may have the potential to overcome some of the reduced spring growth by intercepting more of the available water, as shown at the Ellinbank site (Fig. 5). Breeding or selecting for deeper rooted plants could be an effective means of reducing the effect of climate change in southern Victoria. However, it is unlikely that deeper rooted plant systems could increase production in lower rainfall regions where there is historically little drainage (e.g. the Wagga Wagga site, Fig. 4). Other adaptation options, such as improving the heat tolerance of perennial ryegrass, may also need to be evaluated.
In assessing the effects of these future climate scenarios on pasture production, it is important to recognise the limitations of these scenarios and that the climate change projections will most likely change as global circulation models improve in their description of future climatic systems. The method we used to create the future climate scenarios reflected changes to the mean climate, but does not account for projected increases in extreme climate events, such as heat waves and high-intensity rainfall events (CSIRO and BoM 2007; Alexander and Arblaster 2009). Further quantification of these extreme events is required from climate scientists, before their effects on pasture systems can be adequately modelled. It is important also to recognise that the grazing system models we used do not consider plant persistence, so increased temperature or drought effects on plant mortality were not taken into account. However, it is acknowledged that increased plant mortality and the need to re-sow pastures more frequently could have important management and economic consequences. Finally, there are some uncertainties about responses to elevated CO2; for example, in the Swiss and New Zealand FACE experiments there was evidence of progressive N and phosphorus nutrient limitations developing, and associated increased legume content of pasture (Hartwig and Sadowsky 2006; Newton et al. 2006).
Conclusions
While elevated atmospheric CO2 concentrations alone are likely to increase pasture production, higher temperatures and changes in rainfall patterns will also interact to determine the overall production response under future climate scenarios. In subtropical and subhumid regions of eastern Australia, future climate scenarios indicated warming with little change in annual rainfall, and our study predicted increased pasture production with an extended C4 species growing season. In southern Australia, where future climate scenarios indicated higher temperatures and reduced rainfall, our study showed that this would lead to only small increases in production in the 2030 scenario, but decreases of up to 19% in the 2070 high scenario. Pasture production was predicted to be more resilient to climate change in the cool temperate environment of northern Tasmania. While these analyses reflected the performance of current pasture systems in future climates, there appeared to be some potential in developing adaptation strategies, such as the use of deeper rooted plants in temperate environments, to mitigate the effects of climate change.
Acknowledgments
The Whole Farms Systems Analysis and Tools (WFSAT) project was funded by Dairy Australia, Meat & Livestock Australia, and AgResearch, New Zealand.
Ainsworth EA, Long SP
(2005) What have we learned from 15 years of free-air CO2 enrichment (FACE)? A meta-analytical review of the responses of photosynthesis, canopy properties and plant production to rising CO2. New Phytologist 165, 351–372.
| Crossref | GoogleScholarGoogle Scholar | PubMed |
is a simple Michalis-Menten type response, also referred to as a rectangular hyberbola (Thornley and Johnson 2000). The term in the second parentheses is constant and imposes the constraint Pmx(C = Camb) = Pmx,amb.
The response of plant N level, fN, kg N/(kg dry weight), to CO2 concentrations is described by:

where α, is a curvature coefficient, and KN, ppm, and λ are scaling parameters. According to this equation:

Equation 2a confirms that fN,amb is the value of fN at ambient CO2, while Eqn 2b shows that, when C = KN, fN is the average of the value at ambient and saturated CO2. The third equation in Eqn 2b confirms that fN,amb is reduced by the factor λ at saturated CO2.
Canopy conductance, which is the sum of leaf stomatal conductances in the canopy, declines in response to CO2 as described by:

where β is a curvature coefficient, and gc,mn and gc,mx are values such that:

These simple and versatile functions provide flexibility within the model to explore the consequences of different responses to elevated CO2.