A meta-analysis of plant tissue O2 dynamics
Max Herzog


A The Freshwater Biological Laboratory, Department of Biology, University of Copenhagen, Universitetsparken 4, 3rd Floor, Copenhagen 2100, Denmark.
B Department of Food, Agricultural, Environmental and Animal Sciences, University of Udine, via delle Scienze 206, Udine, Italy.
C School of Agriculture and Environment, The University of Western Australia, 35 Stirling Highway, Perth, WA 6009, Australia.
Functional Plant Biology 50(7) 519-531 https://doi.org/10.1071/FP22294
Submitted: 6 December 2022 Accepted: 13 April 2023 Published: 10 May 2023
© 2023 The Author(s) (or their employer(s)). Published by CSIRO Publishing. This is an open access article distributed under the Creative Commons Attribution-NonCommercial-NoDerivatives 4.0 International License (CC BY-NC-ND)
Abstract
Adequate tissue O2 supply is crucial for plant function. We aimed to identify the environmental conditions and plant characteristics that affect plant tissue O2 status. We extracted data and performed meta-analysis on >1500 published tissue O2 measurements from 112 species. Tissue O2 status ranged from anoxic conditions in roots to >53 kPa in submerged, photosynthesising shoots. Using information-theoretic model selection, we identified ‘submergence’, ‘light’, ‘tissue type’ as well as ‘light × submergence’ interaction as significant drivers of tissue O2 status. Median O2 status were especially low (<50% of atmospheric equilibrium) in belowground rhizomes, potato (Solanum tuberosum) tubers and root nodules. Mean shoot and root O2 were ~25% higher in light than in dark when shoots had atmospheric contact. However, light showed a significant interaction with submergence on plant O2, with a submergence-induced 44% increase in light, compared with a 42% decline in dark, relative to plants with atmospheric contact. During submergence, ambient water column O2 and shoot tissue O2 correlated stronger in darkness than in light conditions. Although use of miniaturised Clark-type O2 electrodes has enhanced understanding of plant O2 dynamics, application of non-invasive methods in plants is still lacking behind its widespread use in mammalian tissues.
Keywords: anoxia, flooding, hypoxia, internal O2, internal oxygen, light, O2 dynamics, oxygen dynamics, submergence, tissue O2, tissue oxygen.
Introduction
Following the discovery of O2 (‘fire air’) in 1771, scientists have attempted to elucidate the interaction of O2 with plants. In photosynthetic organisms, O2 is produced as a by-product of photosynthesis, as electrons removed from H2O (generating O2) pass through PSII and PSI for the generation of NADP(H), subsequently reducing CO2 into organic carbon (Blankenship 2002). Accumulation of O2 inside plant tissues results in a concentration gradient driving a diffusive flux of O2. This flux may be to other parts of the plant (Armstrong 1979), the surrounding environment, or in some specialised wetland plants, even be driven by mass flow into other plant organs (Dacey 1980).
Plant O2 dynamics are important for understanding processes crucial for plant functioning such as respiration, photosynthesis, and reactive O2 species (ROS) production (Blokhina et al. 2003; Gibbs and Greenway 2003; Schmidt et al. 2018). As in most other organisms, O2 in plants acts as electron acceptor in oxidative phosphorylation generating ATP (Millar et al. 2011). When O2 is absent, plants can generate some ATP through glycolysis and anaerobic fermentation, although with much lower ATP yield per molecule of glucose (Bailey-Serres and Voesenek 2008). O2 also leads to photorespiration, where Rubisco reacts with O2 rather than with CO2 to form RuPB in its oxygenase reaction. Photorespiration thus results in carbon loss, but also protects plants from photoinhibition (Kozaki and Takeba 1996). O2 can generate ROS that are important molecules in cell signalling (Van Breusegem et al. 2008), but can also results in cell damage (Blokhina et al. 2003). More recently, the identification of an O2 sensing mechanism in plants (Licausi et al. 2011), with an analogous O2-sensing pathway described for animal tissues (Holdsworth and Gibbs 2020), expanded the knowledge on plant adaptations to low O2. In addition, observations that O2 acts as a signalling molecule in plant development (Weits et al. 2019) has advocated a shift in perceiving low O2 as a plant stressor to also view low O2 as a constitutive, ‘chronic’ condition regulating plant growth and development (Weits et al. 2021).
The occurrence of low O2 status in plant tissues is often overlooked, most likely due to photosynthetic O2 production (Ast et al. 2012). Plants are sessile organisms, which requires them to adapt to environmental changes in their surroundings, including changes in O2 availability (Ast et al. 2012). Flooding has profound effects on the O2 status of plant tissue. Complete or even partial submergence affects plant O2 status due to the 10 000-time slower gas diffusion in water than in air, lower solubility of O2 in water than in air, light attenuation by flood waters and microorganisms depleting flooded soils of O2 (Voesenek et al. 2006). Studies have also demonstrated that biotic and abiotic factors such as pathogen infection, temperature, salinity, and light conditions affect plant tissue O2 status (Kumari et al. 2017; Chung et al. 2019; Koch et al. 2022a, 2022b). Thus, the lack of an active O2 distribution system in plants, presence of non-photosynthetic plant tissues, and a number of biotic and abiotic factors affecting tissue O2, thereby resulting in substantial internal gradients in molecular O2.
Some plants possess mechanisms to control tissue O2 status, such as aerenchyma providing a low resistance pathway for internal O2 transport (Armstrong 1979). Certain plants also show adaptations restricting O2 transport across tissues. For example, O2 diffusion barriers in N2 fixing root nodules limit the diffusive flux of O2 to the root nodule, as O2 would otherwise irreversibly inactivate nitrogenases responsible for N2 reduction (Hunt et al. 1988). Another example of O2 controlling mechanisms is the deposition of lignin and suberin in the cell wall along the outer part of a root restricting O2 loss from roots into anoxic soils (Colmer 2003).
As plant organs consume and produce O2 simultaneously, O2 status within the same plant may vary substantially from organ to organ. For example, in Sacocornia fruticosa, root O2 status was only 39% of the O2 level in photosynthesising shoot tissues (Pellegrini et al. 2017). Moreover, O2 status may vary between adjacent tissues, such as the stele of maize (Zea mays) roots being only 50% of the level in the root cortex over a distance of only 100 μm (Armstrong et al. 1994). Thus, plant tissue O2 status can be expected to be dynamic in space and time, depending on plant organ, tissue type, and environmental conditions.
Over time researchers have used and continue to use contrasting approaches to determine molecular O2 within plant tissues. Data used in this review include the earliest measurements of O2 in plant tissues made using gas analysers, where a discrete gas sample is brought in contact with an absorbent (Thoday 1913; Laing 1940; Scholander 1947; Armstrong and Gaynard 1976; Erdmann and Wiedenroth 1988; Jordan and Whigham 1988), or injected into gas chromatographs (Spalding et al. 1979; Tjepkema and Cartica 1982). More recently, the development of polarographic electrode sensors and O2 optodes resulted in the development of microsensors allowing for O2 measurements with better spatial and temporal resolution than gas sampling (Ast and Draaijer 2014). The development of these sensors has allowed for continuous O2 measurements within tissues, in contrast to discrete measurements obtained by gas sampling.
As evident from the above, plant tissue O2 status is likely to depend on plant organ, tissue type, developmental status, and environmental conditions. In the present study, we aimed at identifying factors controlling tissue O2 status; e.g. important environmental conditions such as light and floods. For this, we extracted 1567 published measurements of vegetative plant tissue O2 levels from the literature spanning 112 plant species, together with other parameters such as ambient O2 levels, light conditions, and flooding status etc. We then conducted quantitative meta-analyses identifying factors controlling tissue O2 status. We depict the overall patterns generated by this vast amount of data, but also highlight different plant strategies in maintaining O2 homeostasis in subsequent, detailed analyses. While including the majority of plant tissues allowing for a general overview, we refer to other studies regarding details on O2 status in seeds (Borisjuk and Rolletschek 2009) and fruits (Ho et al. 2014). We show that plant tissue O2 status depend particularly on the types of plant tissue, light conditions, and plant submergence status. We also show that hypoxic conditions especially develop in dense tissues and some roots, while hyperoxic conditions are observed in submerged, photosynthesising tissues. Finally, we discuss the progress in methods to determine plant tissue O2 status.
Analytical approach
Literature search
We performed a structured and systematic literature search to identify studies reporting O2 measurements in vegetative plant tissues. The search was conducted using the Web of Science BIOSIS database applying Boolean operators, as explained in detail in the Supplementary materials. The search resulted in a final list of 129 studies published up to September 2022 used for this review. We extracted each observation of plant tissue O2 status and entered it into a database alongside information of plant species, tissue type, light conditions, submergence status, treatment, O2 measurement technique, tissue excisions status etc. if provided in the source. The list of studies used for data extraction is available in Supplementary materials. The database of plant tissue O2 levels can be accessed from the Dryad data repository at https://doi.org/10.5061/dryad.cnp5hqc8v.
Data handling
The studies used for this review provide a wide range of ways to measure and report levels of plant tissue O2. The following steps describe how we performed data extraction for our meta-analysis.
O2 levels reported as mm Hg, concentrations (mg L−1, μmol L−1), % of air equilibrium or % O2 was converted into kPa O2 as detailed in Supplementary materials.
When plants were subject to contrasting treatments (submergence, light/dark, pathogen infection etc.), reported values for each separate treatment were extracted.
When tissue O2 was reported as tissue profiles (e.g. through a root cortex) the mean of these data points within each tissue was calculated and used for the analyses. When radial profiles were longitudinally distributed along an organ (stem/root), mean values from each radial profile were extracted separately.
When O2 was reported in a time series (fx diurnally), the time series’ maximum (usually daylight) and minimum (usually late night-time) values were extracted and used for analyses. On some occasions, fluctuating ambient night-time and daytime O2 levels resulted in significant diurnal and/or nocturnal variations in tissue O2 (Pedersen et al. 2016). In these cases, two to three values spread across the period were extracted (early, mid and end of night/day period) and used for the analyses. When O2 was measured over long time series (days, weeks, months) reporting daily/weekly averages, all available data points were extracted (Eklund 2000). In the case of Sorz and Hietz (2008), we extracted all 103 data points but averaged the treatments and seasons.
In a few studies, tissue O2 was sampled by extracting internal plant gasses in a liquid such as ambient flood water. Such studies where we suspected that air bubbles escaping from plants tissues had been in contact with surrounding medium (thus allowing for O2 exchange) prior to measurements were discarded (e.g. Setter et al. (1987); Erdmann and Wiedenroth (1988); Stevens et al. (2002)). Studies where gas sampling was performed using syringes, preventing O2 exchange with the surrounding medium, were included in the analyses.
For correlating submerged plant shoot tissue pO2 with water column pO2, we identified 26 studies simultaneously reporting shoot tissue and water column pO2 levels. Corresponding values of tissue and water column pO2 were then extracted. For diurnal measurement series, two to three values spread across the period were extracted. When water column levels were manipulated, shoot values for each steady state level of water column pO2 were extracted. To allow for effects of internal gas transport to be evident in the meta-analysis, only measurements from intact plants were used for this analysis.
Statistical analysis
Data were analysed using GraphPad Prism 9 (GraphPad Software, La Jolla, CA, USA) or R (R Core Team 2022) for Windows statistical software. Normality of distributions was confirmed by visual inspections of residual plots and Shapiro–Wilk normality test, and homogeneity of variance by using F-test or Brown–Forsythe test (P > 0.05). When data failed these assumptions, data was transformed, or a non-parametric test was chosen. Further details on statistical tests are given in the respective figure captions.
Modelling
We evaluated the influence of environmental conditions, plant species and tissue type on plant O2 status using an information-theoretic model selection approach. This explorative, data-driven approach is increasingly used when several predictors may be associated with a particular trait (Symonds and Moussalli 2011). The information-theoretic model selection approach differs from traditional null-hypothesis significance testing, where a hypothesis is first defined, and then accepted or rejected based on P-values (Symonds and Moussalli 2011). Each competing model predicts the outcome variable (in our case: plant tissue O2 status) using predictors and their interactions (in our case, submergence, light, tissue type etc.). Thus, each competing model can be viewed as a different hypothesis of how predictors affect plant O2. Ranking the competing models allowed us to estimate which model best explains tissue O2 status. We used a type of regression analysis, Linear Mixed-effects Model (LMM), to estimate the model parameters and their importance in controlling plant O2 status. The use of mixed model allowed us to include plant species as a random factor, taking the variability in plant tissue O2 related to species specific factors (e.g. aerenchyma formation) into account.
We compared all the possible predictors and combinations of interaction and selected the best model using the Akaike Information Criterion (AIC) (Burnham and Anderson 2004). AIC values derive meaning from comparing with AIC of other generated candidate models. By penalising the number of model predictors, AIC allows for the selection of the ‘best’ model based on fit (e.g. large R2) but also model complexity, and thus presents a compromise between fit and complexity. For details on applied R packages, data categorisation and diagnostic plot (Fig. S1), see Supplementary materials.
Results and discussion
Our comprehensive analysis of 129 studies on 112 plant species, including 1567 reported tissue O2 measurements, showed that tissue O2 status ranged from anoxia1 to more than 2.5-fold atmospheric equilibrium. Our data-driven model selection identified ‘submergence’, ‘light’, ‘tissue type’ and ‘light × submergence’ as key parameters affecting plant tissue O2 status, and we further conducted detailed meta-analyses allowing us to quantify the effects of ‘submergence’, ‘light’, and ‘tissue type’ on tissue O2 status.
‘Submergence’, ‘light’, and ‘tissue type’ significantly affect plant tissue O2 status
Our information-theoretic model selection identified ‘submergence’, ‘light’, ‘tissue type’ and ‘light × submergence’ interaction as the most important predictors of plant tissue status; i.e. these predictors had a relative variable importance of 1.0 in the only plausible linear mixed-effects model (Table 1). Meanwhile, the ‘tissue type × light’ interaction only had a relative variable importance of 0.8. This indicates that the ‘tissue type × light’ interaction was a weaker predictor of tissue O2 than the other predictors.
Model number | Model dependent variable | Intercept | Submergence | Light | Tissue type | Light × submergence | Tissue type × light | df | R2C | R2m | AIC | ΔAIC | Weight |
---|---|---|---|---|---|---|---|---|---|---|---|---|---|
Model 48 | Sqrt(pO2) | 3.351 | + | + | + | + | + | 12 | 0.5485 | 0.4030 | 3198 | 0.00 | 0.694 |
RVI | 1.0 | 1.0 | 1.0 | 1.0 | 0.8 | – | – | – | – | – | – | ||
Model 16 | Sqrt(pO2) | 3.330 | + | + | + | + | – | 10 | 0.5390 | 0.3983 | 3201 | 2.75 | 0.176 |
RVI | 1.0 | 1.0 | 1.0 | 1.0 | – | – | – | – | – | – | – |
The two best models are shown, but only model 48 was statistically plausible (ΔAIC < 2). The models explained tissue O2 status in relation to predictors ‘light’ (i.e. light or dark conditions), ‘submergence’ (i.e. shoot completely submerged, partially submerged, or shoot completely in air), ‘tissue type’ (root, stem or leaf) and interactions. Only studies clearly describing light, submergence and tissue types were included (101 studies). Data from experiments where tissues were excised or the O2 level of the atmosphere/submergence medium around the shoot was manipulated (i.e. atmosphere or submergence solution around shoot was purged with N2) was excluded. This filtering resulted in the inclusion of data from 92 species, and ‘plant species’ was included as random factor. Tissue O2 partial pressures (pO2) were square root transformed prior to the analysis. Intercept, df, marginal R2m (variance explained by fixed factors) and conditional R2c (variance explained by both fixed and random factors), Akaike information criterion (AIC) and ΔAIC values, model weight (Weight) and relative variable importance (RVI) are reported. ‘+’ indicates if a predictor was included in the respective model.
Including species as a random factor increased the model fit. This is evident as marginal R2 was 0.40 without species as a random factor, compared with conditional R2 of 0.55 with the random factor included (Table 1). Following the data-driven identification of the predictors ‘submergence’, ‘light’, ‘tissue type’ and ‘light × submergence’ controlling plant tissue O2 status, we continued by exploring our database of published O2 status for patterns generated by these predictors.
Plant O2 status depends on plant tissue type
Our meta-analysis showed that O2 status declined significantly from leaves to stems, and from stems to roots; i.e. median O2 status was 15.7 kPa in leaves, 13.1 kPa in stems and 9.8 kPa in roots, respectively (Fig. 1). We interpret the significantly higher O2 status in leaves to be caused in three ways: (1) by leaves producing endogenous O2 via photosynthesis when in light; (2) by leaf stomata being able to vent leaf gas spaces, thus allowing for leaf O2 status to remain closer to atmospheric levels than in more dense tissues without stomata; and (3) by leaves normally being in close contact with the atmosphere, in contrast to roots or rhizomes, which are in direct contact with O2 consuming soils or sediments. Indeed, violin-plots showed a relatively high number of anoxic conditions in root tissues compared with leaves (Fig. 1). It should be noted that distribution of O2 levels in roots may be influenced by a high number of wetland and aquatic plants in the published data underlying this analysis. In detail, 78% of the observations originated from aquatic and wetland plant species and 21% from terrestrial plants. Plant species inhabiting wetland and aquatic systems possess root traits such as aerenchyma that enhance internal aeration and thus ensure adequate tissue O2 supply for root functioning, whereas terrestrial plants typically lack such traits leading to O2 depletion in flooded soils (Armstrong 1979). The distribution of O2 levels reported in Fig. 1 should therefore be treated with caution when inferring a ‘true’ distribution of O2 in all plants.
O2 partial pressures (pO2) in leaf, stem and root tissues. Numbers of replicate values (n): 322, 633 and 404 for leaf, stem, and root tissues, respectively. Letters denote significant difference between medians (non-parametric Kruskal–Wallis test with Dunn’s multiple comparisons, P < 0.05). Truncated violin plot shapes illustrate observation frequency, the solid line the median and dashed line the quartiles. Data from experiments where the O2 level of the atmosphere/submergence medium around the shoot was manipulated (i.e. atmosphere or submergence solution around shoot was purged with N2) was excluded in this figure.
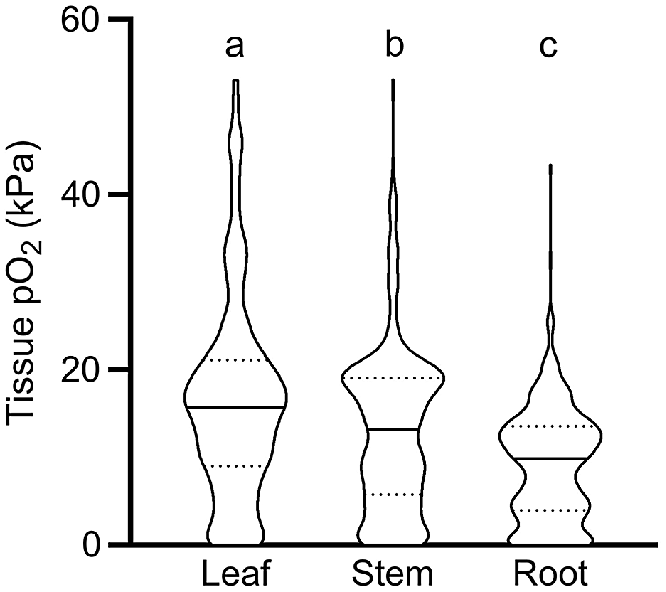
The broad categorisation of tissue type into leaf, stem and root in Fig. 1 does not do justice to the vast range in morphology and function of different tissue types within these main categories. For example, the category of roots encompasses diverse tissues such as root tips in anoxic soils, mangrove pneumatophores (acting as pathways for atmospheric O2 inlet), aquatic adventitious roots and other contrasting root tissues. For example, two very high root tissue O2 levels (43.2 and 32.5 kPa, Fig. 1) were from chlorophyll containing, photosynthesising aquatic adventitious roots of Meionectes brownii (Rich et al. 2013). In addition to the broad categories in Fig. 1, we therefore also categorised measurements from leaf, shoot and root tissues into several subcategories (Fig. 2).
O2 partial pressures (pO2) in (a) leaf, (b) stem and (c) root tissue. In (b) ‘Stem tissues’ encompass stems, culms, stem internodes, peduncles, or stolon tissues but not rhizomes, storage organs (tubers and corms) and woody tree stems. Tree stems are woody stems of beech (Fagus sylvatica), Norway spruce (Picea abies), olive (Olea europaea), red maple (Acer rubrum), eastern hemlock (Tsuger canadenis), Northern red oak (Quercus rubra), black poplar (Populus nigra) and American ash (Fraxinus americana). Numbers of replicate values (n): leaf blade/lacunae (102), leaf sheath (13), leaf petiole (125), flower (11), leaf/flower bud (14), ‘Stem’ (306), tree stem (97), potato tubers (28), rhizome (135), root axis (320), aquatic roots (8), pneumatophore (9), root nodule (15). Letters denote significant difference between medians (non-parametric Kruskal–Wallis test with Dunn’s multiple comparisons, P < 0.05). Truncated violin plot shapes illustrate observation frequency, the solid line the median and dashed line the quartiles. Data from experiments where the O2 level of the atmosphere/submergence medium around the shoot was manipulated (i.e. atmosphere or submergence solution around shoot was purged with N2) was excluded in this figure.
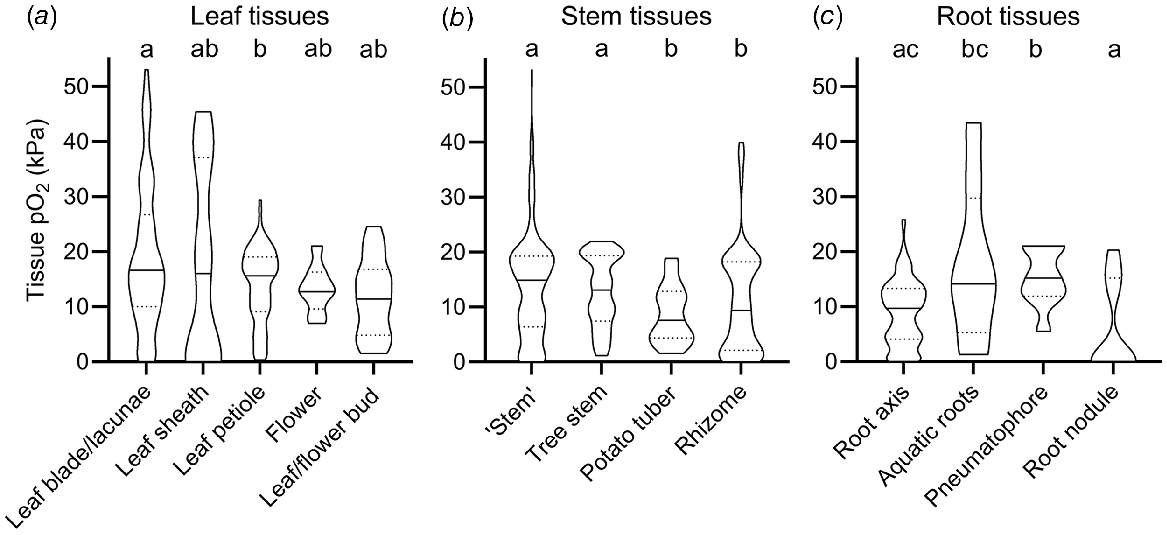
In the leaf tissues subcategories O2 levels were relatively similar compared with the vast variation in stem and root tissues; i.e. median O2 status only ranged from 11.4 to 16.8 kPa (Fig. 2a). The only significant difference was found between median leaf blades (16.8 kPa) and leaf petioles (15.2 kPa). There was no significant difference between the remaining leaf categories (leaf sheath, flower, and bud tissues), most likely due to a low number of O2 observations in these tissues (n < 14) compared with leaf blades and petioles (n > 102).
Tissue O2 status of stems showed larger variation than leaf tissue and was significantly influenced by stem tissue type. Hence, O2 status in stem tissues was lower in thick, belowground, and predominantly respiring tissue types, compared with porous and photosynthesising tissue types (Fig. 2b). In stems, median O2 status in tissues such as culms, peduncles, stolons and stems of herbaceous plants (14.9 kPa, designated as ‘stems’ in Fig. 2b) and tree stems (13.1 kPa) were significantly higher, than in rhizomes (9.4 kPa) and the dense storage organ of Solanum tuberosum (7.6 kPa, designated as potato tuber in Fig. 2b). Thick and dense tissues showing high resistance across the diffusion path are prone to internal O2 gradients, especially if respiratory demand for O2 is large (Armstrong 1979; Colmer and Greenway 2005). Indeed, 32 g potato tubers experienced relatively low central O2 status of 1.5 kPa compared with 7 kPa in 8 g small tubers (Geigenberger et al. 2000). Another example of low internal O2 status in dense stem tissues results from radial profiling across dense succulent stem tissues of submerged Halosarcia pergranulata using an O2 microsensor (Pedersen et al. 2006). Here, internal O2 declined to 1.5 kPa compared with 21 kPa in the submergence solution. Respiration rates also affect plant tissue O2, exemplified in potatoes stored at 22–25°C showing less than half of the tissue O2 compared with potatoes stored at 5–11°C (Stiles 1960). In summary, stem tissue O2 was particularly low in thick, dense, or predominantly respiring tissues such as succulent tissues or belowground rhizomes.
In stark contrast to potato tubers and succulent stems, median O2 was significantly higher in the other stem categories. Plant stems may contain lysigenous or schizogenous aerenchyma, facilitating gas exchange via interconnected air spaces that enhance O2 diffusion due to the 10 000-fold higher diffusion coefficients in gas than in liquid phase (Armstrong 1979). Since diffusion is a very slow process over long distances, some specialised wetland plants can induce ‘internal winds’ (pressure driven, convective gas flow) within connected air spaces allowing for effective O2 transport over long distances (Dacey 1980). For example, O2 in submerged culms of the sedge Eleocharis sphacelata remained close to atmospheric levels of 20 kPa down to 2.7 m of water depth during daytime where convective flow was operating, compared with <10 kPa during the night where convective flow is absent (Sorrell and Tanner 2000). This was a result of pressurised flow of gas from influx culms to efflux culms of up to 5.8 mL min−1, supplying immersed tissues with atmospheric O2.
Root tissue O2 showed even higher range in median O2 status than leaf and stem tissues. Median O2 reached 15.2 kPa in pneumatophores but was 0 kPa in root nodules (Fig. 2c). In root nodules, symbiotic bacteria convert N2 gas into ammonia to be used in amino acid biosynthesis. Since the process of nitrogen fixation is highly O2 sensitive, root nodules have evolved mechanisms such as leghaemoglobin to regulate free O2 inside the nodule (Downie 2005). Indeed, the few observations of significant O2 inside root nodules belong to experiments where nodules were inoculated with mutant bacteria strains, some of which were unable to infect plant cells (Masepohl et al. 1993; Romanov et al. 1995). In contrast, the median O2 level in pneumatophores was significantly higher (15.2 kPa) than in the root axis or root nodules. Pneumatophores are lateral roots known from plants growing in anoxic mudflats such as mangroves (e.g. Avicennia marina), where they exhibit negative geotropism thereby growing upward. When reaching the sediment surface, O2 can pass through small bark openings (lenticles) and diffuse into the belowground root system. Other root tissues showing high O2 status were photosynthesising aquatic roots (Rich et al. 2011). Here, O2 status were as high as 43.2 kPa (median of 14.2 kPa, Fig. 2c). O2 may build up to such high levels in submerged, photosynthesising tissues due to physical restrictions in gas diffusion preventing photosynthetic O2 to diffuse out of the tissues and into the surrounding water.
O2 gradients within plant tissues
Obtaining O2 status with high spatial resolution has also revealed that O2 status vary significantly not only between but also within tissue types. Such data has mainly been obtained using Clark-type O2 microelectrodes. With a tip diameter of down to 3 μm (Weits et al. 2019) the electrode can be positioned in delicate tissues such as roots, even in field conditions. Our meta-analysis of literature data from studies reporting 40 paired root measurements of stele and cortex O2 status, reveal that mean O2 status in the root stele was 66% of the cortex O2 status (Fig. 3). The root stele is known to be more metabolically active compared with cortex tissues; e.g. for banana (Musa spp.) roots, a 6-fold difference in respiration rate was found (Aguilar et al. 2003). In addition to displaying high respiration rates, stele tissues only contain relatively few air spaces that further increases the O2 demand per volume of root tissue. In contrast, the root cortex may contain large air spaces caused by aerenchyma formation, allowing for longitudinal flow of O2 from the shoot or atmosphere toward the root tip (Armstrong 1979). Thus, the resistance to O2 diffusion increases in the dense stele compared with the cortex. The high respiration rates and low diffusivity in dense tissues result in the lower O2 status within root steles, as supported by modelling results (Armstrong et al. 2000).
O2 partial pressures (pO2) in root cortex and stele tissues reported in the literature. Values were extracted from 13 published studies where O2 microsensors were driven through both tissues (i.e. matched pairs). * denotes statistical significant difference between means (paired t-test on square-root transformed data, P < 0.0001, n = 40). Truncated violin plot shapes illustrate observation frequency, the solid line the median and dashed line the quartiles. Mean pO2 was 6.2 kPa in the cortex and 4.1 kPa in the stele, respectively.
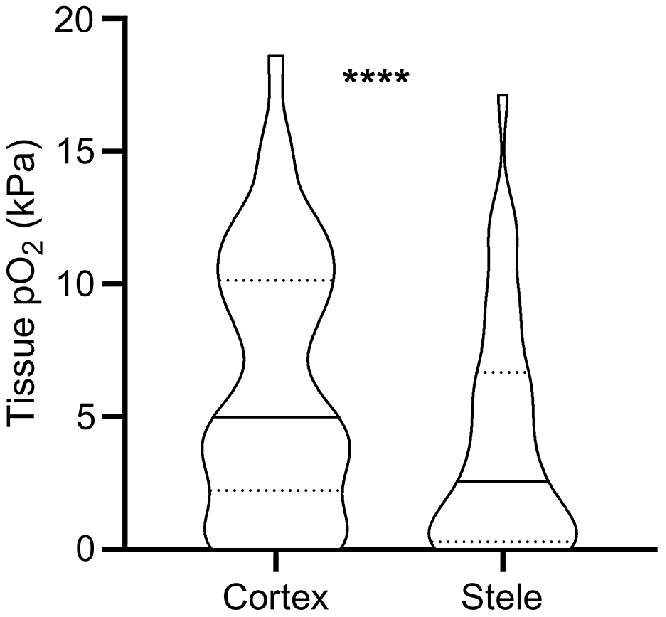
In addition to root tissues, shoot tissues may also exhibit internal O2 gradients. Molecular O2 inside the shoot apical meristem of Arabidopsis thaliana (3.6–8.4 kPa) compared with the surrounding stem tissue (13–19 kPa) regulated leaf production, with hypoxia inhibiting proteolysis of an N-degron-pathway (Weits et al. 2019). The observed gradient in the shoot apex of 15 kPa developed over a distance less than 50 μm. Meanwhile, O2 within the 2-mm thick leaf petioles of Rumex palustris was much more homogeneous, probably owing to the large number of internal gas spaces (aerenchyma content of >20%) and underwater photosynthesis of the green petioles (Mommer et al. 2004). Corresponding to a longitudinal decline of O2 in roots (discussed below), profiles along the leaf petioles of R. palustris exhibited ~3 kPa longitudinal O2 decline from a position close to the O2 source (leaf) to O2 sink (roots) (Mommer et al. 2004).
Plant tissues also show significant longitudinal variation in internal O2 status. In roots, the degree of longitudinal O2 diffusion from the shoot (O2 source) via the root base to the root tip (O2 sink) is crucial for plant functioning when roots grow in O2 free media such as soils with excess water (Armstrong 1979). Plant adaptations and acclimation enabling a continuous supply of shoot-derived molecular O2 to the metabolically active root tip have therefore been thoroughly studied. Key traits such as aerenchyma formation (constitutive as well as inducible) and induction of barriers to radial O2 loss, which prevent O2 leaking from the root axis into anoxic soils, allow for continued root aeration despite the anoxic soil environment (Colmer 2003). To evaluate the effect on root aeration of a barrier to radial O2 loss and aerenchyma formation, one can compare O2 status of roots grown in a stagnant, deoxygenated nutrient solution mimicking a flooded soil (Wiengweera et al. 1997) with roots grown in aerated nutrient solution (mimicking a drained soil). Most wetland species will further induce aerenchyma and a barrier to radial O2 loss only in the former condition. For example, in Zea nicaraguensis, root apical O2 status was 8.7 kPa in roots formed in stagnant, deoxygenated nutrient solutions compared with 0.47 kPa in roots from aerated nutrient solution when measured in roots in a severely hypoxic solution (Pedersen et al. 2021). In the roots formed in stagnant, deoxygenated nutrient solution, a barrier to radial O2 loss was induced, and root aerenchyma was 1.4–fold of that under aerated conditions (Pedersen et al. 2021). Thus, in this concrete example, aerenchyma and the barrier to radial O2 loss resulted in better O2 status of the roots formed in the stagnant, deoxygenated nutrient solution.
In order to isolate the effect of the barrier to radial O2 loss on root aeration without shoot-derived O2 and varying root lengths impeding interpretations, a recent study reported radial O2 intrusion into submerged root segments of rice (Oryza sativa) (Peralta Ogorek et al. 2021). Here, maximum O2 status within the root segments with a weak barrier to radial O2 loss differed 54-fold from roots with a tight barrier, when incubated with 21 kPa O2 in the surrounding water (Peralta Ogorek et al. 2021). The strong effect of the barrier to radial O2 loss on root O2 status underlines the importance of species-specific adaptations and/or acclimations for O2 status in plant tissues.
In conclusion, both root and shoot tissues may show substantial radial and longitudinal internal O2 gradients. The gradients depend on tissue density, respiration activity, plant adaptations and/or acclimations such as aerenchyma and radial O2 loss barrier formation, and environmental conditions.
O2 status in submerged plant tissues – the importance of light
Submergence is a compound stress to plants, brought on by several effects. For one, the 10 000–fold slower gas diffusion in liquid compared with gas impedes the diffusive supply from the environment of O2 for respiration and CO2 for photosynthesis (Jackson and Drew 1984). As floodwaters absorb and scatter incoming light, photosynthesis may be hampered even further (Colmer et al. 2011). Build up of the volatile phytohormone ethylene may induce leaf senescence as chlorophyll is degraded (Voesenek and Bailey-Serres 2015). Thus, the capacity for underwater photosynthesis and therefore O2 production generally declines with time of submergence for terrestrial vegetation (Colmer et al. 2011). Indeed, our data-driven model-selection approach identified both ‘submergence’ and ‘submergence × light’ interaction as factors that significantly affect plant tissue O2 status (Table 1). It should be noted that the identification of ‘submergence’ over other environmental conditions could be due to a relatively large number of studies focusing on plant tissue O2 in submerged plants (52% of observations used for model selection), compared with plants subject to drought, nutrient limitations, or pathogen infections. Nevertheless, the effect of submergence on plant tissue O2 per se was validated by the model selection. To further quantify the effect of light and submergence on plant tissue O2 status, we filtered data from the literature where O2 was measured inside intact plants during complete submergence or with atmospheric contact (drained with shoot in air, waterlogged with shoot in air, or partial submerged shoot), and in light or dark conditions. We excluded data from experiments where the O2 level of the atmosphere/submergence medium around the shoot was manipulated (i.e. atmosphere or submergence solution around the shoot was purged with N2), as this would otherwise mask any light or submergence effects. This analysis underlined the vast importance of light particularly for shoot tissues (Fig. 4). Shoots in air contained 24% less O2 in dark than in light, most likely owing to the lack of photosynthetic O2 under dark conditions. The effect of light on tissue O2 was also evident in root tissues, where mean O2 status declined from 10.6 kPa in roots of plants in light to 7.9 kPa in roots of plants under dark conditions.
O2 partial pressures (pO2) in (a) shoot tissues and (b) root tissues during complete submergence (‘shoot submerged’, filled bars) or the shoot in air (‘shoot in air’, i.e. drained conditions, waterlogging and partial submergence, open bars) in light or dark conditions. Data were extracted from 117 studies where O2 was measured inside intact plants (i.e. excluding studies where O2 was measured within excised tissues) and where the shoot was clearly stated as being in light or dark conditions. Data was excluded when the O2 level of the atmosphere/submergence medium around the shoot was manipulated (i.e. atmosphere or submergence solution around the shoot was purged with N2). (a) Letters denote statistically significant differences between means (P < 0.05, Tukey post hoc test; n = 201, 226, 106 and 221 for columns from left to right) following 2-way ANOVA showing a significant light (P < 0.0001) and submergence × light interaction (P < 0.0001). (b) Letters denote statistically significant differences between means (P < 0.05, Tukey post hoc test; n = 115, 48, 56 and 31 for columns from left to right) following 2-way ANOVA showing a significant light (P < 0.0001), submergence (P = 0.0001) and submergence × light interaction (P = 0.0078). Bars show mean and error bars s.e.m.
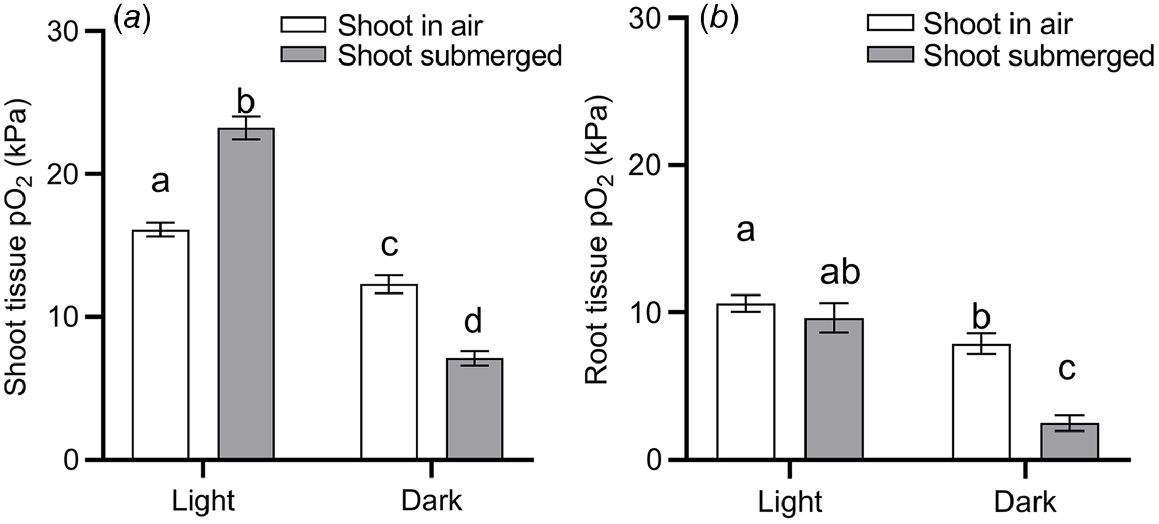
The effect of complete submergence on shoot tissue O2 status was strongly light dependent. In the presence of light, submergence resulted in a significant increase (44%) in shoot tissue O2 status, compared with shoots with air contact (Fig. 4a). In contrast, when the entire shoot was submerged in dark conditions, submergence resulted in a significant decline (42%) in O2 status. Hence two-way ANOVA showed a significant ‘light’ and ‘submergence × light’ interaction. We interpret the submergence-induced increase of shoot tissue in light as a build up of O2 deriving endogenously from underwater photosynthesis, or to a lesser extent, from floodwaters containing high levels of O2. O2 produced in shoot tissues by underwater photosynthesis is likely to build up as stomatal closure, physical boundary layers and slow outward diffusion of O2 into the aquatic environment hampers gas exchange between the shoot and its surroundings (Sand-Jensen et al. 2005; Pedersen et al. 2006, 2016). In contrast, in shoots with atmospheric contact, O2 deriving from photosynthesis would be vented into the surrounding air. The importance of light on plant tissue O2 in situ is nicely exemplified by diurnal leaf O2 and irradiance measurements in seagrass (Thalassia testidinum) (Koch et al. 2022a). Here, a decline in daytime irradiance of ~1000 μmol photons m−2 s−1 from passage of clouds resulted in a rapid decrease in leaf O2 status from 45 to 27 kPa.
The submergence-induced increase in shoot tissue O2 observed in Fig. 4a could also be due to high levels of O2 in floodwaters. High levels (>30 kPa) of O2 in natural floodwaters have been observed in marine (Pedersen et al. 2016) and freshwater (Rich et al. 2013) ecosystems. To test how floodwater O2 levels affect plant tissue O2, we performed a correlation analysis of data from studies simultaneously measuring plant and floodwater O2 levels (Fig. 5). Correlation between shoot tissue O2 levels and water column O2 levels was significant in both cases but stronger in the dark (r = 0.6808, Spearman, P < 0.0001) than in light (r = 0.1925, Pearson, P = 0.0384). The weaker correlation in light than in the dark indicates that production of O2 in underwater photosynthesis provides the plant with an alternative O2 source. As net underwater photosynthesis produces excess endogenous O2, the plant is less likely to experience the same O2 level as the surrounding water column. In contrast, plants submerged in the dark rely on their immediate surroundings as O2 source, and hence the correlation is stronger under dark conditions than in the light. Since plant respiration consumes O2, points cluster below the line of equality in the dark (Fig. 5), but not in light where photosynthesis can elevate tissue O2 to above water column O2.
O2 partial pressures (pO2) in submerged tissues vs O2 levels in the surrounding water column under (a) dark or (b) light conditions with the line of equality (identity line). Values were extracted from 26 studies. O2 values are from freshwater plants (Lobelia dortmanna, Litorella uniflora and Isoetes autralis), seagrasses (Cymodocea nodosa, Thalassia testudinum, Zostera marina, Zostera muelleri, Syringodium foliforme, Posidonia sinuosa, Thalassia hemprichii and Enhalus acoroides) and wetland plants (Suaeda maritima, Glyceria fluitans, Rumex palustris, Halosarcia pergranulata, Meinocetes brownii, Spartina anglica and Oryza sativa) where O2 in submerged tissues was measured simultaneously with water column O2. In experiments where diurnal variations were recorded, diurnal minimum and maximum values were extracted. For Pedersen et al. (2016), tidal waters caused the water column O2 to fluctuate so O2 measurements at the start, middle and end of the dark and light period, respectively, were extracted. Tissue types were leaves, leaf sheaths, rhizomes, stems, leaf meristems and leaf petioles but not roots. (a) Tissue and water column pO2 recorded under dark conditions (at night in field experiments, or without day light/artificial light in laboratory experiments). Spearman correlation coefficient = 0.6808, P < 0.0001 (n = 325). (b) Tissue and water column pO2 recorded under light conditions (during daytime in field experiments, or with artificial light in laboratory experiments). Pearson correlation coefficient = 0.1925, P = 0.0384 (n = 116).
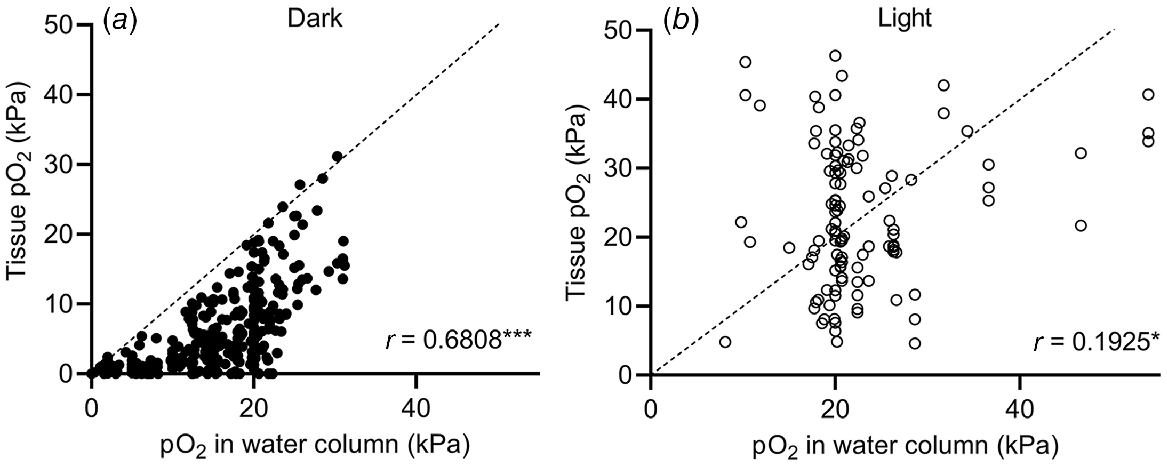
Interestingly, three measurements from the leaf lacunae of the small isoetid Lobelia dortmanna (Møller and Sand-Jensen 2011) showed anoxic conditions in the shoot but high levels of floodwater O2 (21–22 kPa) (Fig. 5). The reason why floodwater O2 did not diffuse into the leaves of L. dortmanna most likely relates to its strategy for CO2 acquisition. L. dortmanna is found in oligotrophic soft water lakes, where underwater photosynthesis is often carbon limited. Thick leaf cuticles therefore reduce permeability to CO2 (and O2), helping L. dortmanna to acquire sediment-derived CO2 for underwater photosynthesis via its root system. In the experiments by Møller and Sand-Jensen (2011), the sediment was enriched with organic matter, resulting in sediment O2 depletion. Due to the lack of a barrier to radial O2 loss in the root, and continuous internal air lacunae, O2 was readily lost from the plant to the sediment. Meanwhile, the thick leaf cuticle restricted O2 exchange with oxygenated floodwater. These observations nicely underline how plant tissue O2 dynamics follow general trends but may deviate if habitats require special adaptations.
Continuous O2 measurements using microelectrodes have revealed severe hyperoxia of >40 kPa O2 (Sand-Jensen et al. 2005; Pedersen et al. 2006, 2016; Rich et al. 2013; Koch et al. 2022a) in submerged wetland and aquatic plants. The highest non-manipulated plant tissue O2 level reported in the literature (53.1 kPa) was measured in Thalassia hemprichii subjected to submergence by tidal waters and high radiation (2000 μmol photons m−2 s−1) (Pedersen et al. 2016). Hyperoxia in submerged vegetation coincides with high irradiation and may even intermit with night time hypoxia (Sand-Jensen et al. 2005; Pedersen et al. 2006, 2016). High levels of O2 may result in the formation of ROS and increased photorespiration; however, the degree of tissue damage and plant mechanisms to tolerate hyperoxia remain much less studied than for hypoxia.
In our meta-analysis of tissue O2 status, we have attempted to summarise the current state of knowledge on plant O2 dynamics. Much knowledge on plant tissue O2 dynamics has been gained due to ongoing development of new O2 measuring techniques. In the following, we therefore present an overview of the progress in determining plant tissue O2 status. We then use this overview to identify future opportunities to obtain further insight of plant O2 dynamics.
Measuring O2 in plant tissues: past, present, and future approaches
The earliest measurements of O2 in plant tissues made use of various forms of gas analysers. Gases sampled from tissues using syringes (or by crushing in mercury) was drawn into a glass chamber, which was then brought into contact with an O2 absorbing solution, allowing for the decrease in gas volume to be monitored (Thoday 1913; Scholander 1947). The earliest methods to our knowledge was the Bonnier-Mangin microtechnique (Thoday 1913) with adaptations by Scholander (1947), used as late as 1994 on tomato (Solanum lycopersicum) and potato tubers (Nery and Calbo 1994). Other studies (Laing 1940) applied the Henderson-Haldane gas analyser, widely used in human physiology research, for measuring O2 in aquatic plants. Finally, gas sampled from tissues with syringes has been analysed using gas chromatographs (Spalding et al. 1979; Tjepkema and Cartica 1982). To our knowledge, the results obtained using gas analysers have not been subject to a direct comparison with other methods such as microsensors.
The development of microsensors allowed for O2 measurements with improved spatial and temporal resolution (Revsbech 1989). The most popular sensors are miniaturised, stiff and narrow (down to 3 μm tip diameter) Clark-type sensors, ideal for penetrating plant tissue and measuring O2 within plants (Pedersen et al. 2020). Optical O2 sensors (O2 optodes) can also be positioned within plant tissues, especially if built into a syringe. Foils coated with O2-sensitive fluorescent dyes and optical O2 sensor nanoparticles are often used for mapping O2 on plant surfaces and their surroundings (Rudolph et al. 2012; Brodersen et al. 2014; Koren et al. 2015). However, optical O2 sensor nanoparticles have only seen limited application inside plants tissues due to their size of up to 600 nm (Schmälzlin et al. 2005; Shaw and Honeychurch 2022). Because of microsensor popularity in plant research, >70% of the 1567 observations of plant tissue O2 used in this study were obtained using an O2 microsensor. For a more detailed review on use of microsensors in plant biology, see Pedersen et al. (2020).
While the abovementioned methods allow for direct O2 measurements within plant tissues, more indirect methods have also been applied. Measurements of radial O2 loss from roots using cylindrical platinum electrodes have been used to model root internal O2 status (Armstrong and Wright 1975); see also Jiménez et al. (2021) for a recent review on methods on measurement of radial O2 loss from roots. Another indirect measurement of tissue O2 status is related to the expression of genes strongly upregulated upon low O2 (referred to as hypoxic reporters) (Mustroph et al. 2010).
In the present meta-analysis, we identified ‘tissue type’, ‘submergence’, ‘light’ and ‘light × submergence’ interaction as important environmental factors for plant O2 status. When conducting meta-analysis, researchers should consider the risk of ‘publication bias’, a term originating from health sciences describing information suppression mechanisms (Dickersin 2005). This includes language bias or accession bias (selective inclusion of studies that are accessible, and published in English), and outcome bias (selective reporting of statistically significant results) among others (Dickersin 2005). The latter would translate to studies reporting surprisingly low or high plant tissue O2 levels, or significant treatment effects of for example plant submergence, being more likely to reach publication than negative results, either due to bias at the investigator or editorial level. The concern that O2 measurements of experiments revealing no treatment effects are under-reported cannot be excluded. However, considering the large number of studies across time, space, species, and methodology describing significant effects of light and submergence on plant tissue O2 levels, we consider their potential importance as validated by this study. We also believe that our data gives an impression of the range and variability in plant tissue O2 levels, although random sampling both at community, species and tissue level would be required to achieve a distribution resembling the ‘true’ distribution of O2 in all plants.
Methods to conduct non-invasive O2 measurements at high spatial and temporal resolution should promote expansion of plant tissue O2 research from predominantly studying the effect of submergence into other areas. Areas already under investigation include studying plant O2 levels in relation to plant development (Weits et al. 2019), pathology (Kumari et al. 2017) and plant signalling (Weits et al. 2021). It has been noted that unravelling tissue O2 dynamics in plants still lacks behind the mammalian field (Ast et al. 2012; Schmidt et al. 2018). This is underlined by the search term ‘tissue hypoxia’ in the Web of Science BIOSIS Previews database resulting in 34 970 records belonging to the ‘Mammalia’ taxa compared with 1041 in ‘Plantae’ (performed in November 2022). In mammals, cancer research resulted in the development of methods such as positron emission tomography (PET), genetically encoded sensors, and 18F NMR for non-invasive O2 measurements (Schmidt et al. 2018). Challenges in translating these methods to plant biology include plant tissue autofluorescence (Ast et al. 2012). However, PET scans of plants tissues used to study carbon dynamics illustrate the possible future application of this technique in plant science (Mincke et al. 2021).
Conclusion
Based on the extraction of 1567 recorded tissue O2 levels from 112 plant species, we found that plant tissue O2 status differs significantly between tissue types. In general, average O2 status is lower in non-photosynthetic and belowground tissues such as roots (9.8 kPa) and rhizomes (9.4 kPa), compared with the leaf lamina (16.8 kPa) and stems (14.9 kPa). However, exceptions exist as exemplified by photosynthesising aquatic roots and mangrove pneumatophores showing relatively high O2 status compared with other root tissues. Environmental conditions that significantly affected plant O2 status were light conditions and submergence. Interestingly, light interacted significantly with submergence on plant tissue O2 status so that while submergence under light caused shoot O2 status to increase by 44% compared with non-submerged shoots, submergence in the dark reduced shoot tissue O2 by 42%. Thus, restricted gas exchange during submergence in light caused shoot tissues to accumulate photosynthetic O2, while the O2 status declined towards hypoxic conditions upon submergence in the dark. Tissue O2 under submergence correlated stronger with floodwater O2 in dark than in light, where shoot tissues could photosynthesise and thus render plant tissues less dependent on floodwater O2. While the development of O2 microsensors has resulted in increased insight into plant tissue O2 dynamics, the field lacks behind the knowledge of O2 dynamics in mammalian tissues. We therefore predict that the use of non-invasive techniques in the future will shed further light on plant tissue O2 dynamics.
Data availability
The data that support this study are available in Dryad at https://doi.org/10.5061/dryad.cnp5hqc8v.
Acknowledgements
We thank Lucas León Peralta Ogorek for comments on a draft manuscript and Kenneth Thorø Martinsen for statistical advice. We also thank the two anonymous reviewers for constructive criticism of this manuscript.
References
Aguilar EA, Turner DW, Gibbs DJ, Armstrong W, Sivasithamparam K (2003) Oxygen distribution and movement, respiration and nutrient loading in banana roots (Musa spp. L.) subjected to aerated and oxygen-depleted environments. Plant and Soil 253, 91-102.
| Crossref | Google Scholar |
Armstrong W, Gaynard TJ (1976) The critical oxygen pressures for respiration in intact plants. Physiologia Plantarum 37, 200-206.
| Crossref | Google Scholar |
Armstrong W, Wright EJ (1975) Radial oxygen loss from roots: the theoretical basis for the manipulation of flux data obtained by the cylindrical platinum electrode technique. Physiologia Plantarum 35, 21-26.
| Crossref | Google Scholar |
Armstrong W, Strange ME, Cringle S, Beckett PM (1994) Microelectrode and modelling study of oxygen distribution in roots. Annals of Botany 74, 287-299.
| Crossref | Google Scholar |
Armstrong W, Cousins D, Armstrong J, Turner DW, Beckett PM (2000) Oxygen distribution in wetland plant roots and permeability barriers to gas-exchange with the rhizosphere: a microelectrode and modelling study with Phragmites australis. Annals of Botany 86, 687-703.
| Crossref | Google Scholar |
Ast C, Schmälzlin E, Löhmannsröben H-G, Van Dongen JT (2012) Optical oxygen micro- and nanosensors for plant applications. Sensors 12, 7015-7032.
| Crossref | Google Scholar |
Bailey-Serres J, Voesenek LACJ (2008) Flooding stress: acclimations and genetic diversity. Annual Review of Plant Biology 59, 313-339.
| Crossref | Google Scholar |
Blokhina O, Virolainen E, Fagerstedt KV (2003) Antioxidants, oxidative damage and oxygen deprivation stress: a review. Annals of Botany 91, 179-194.
| Crossref | Google Scholar |
Borisjuk L, Rolletschek H (2009) The oxygen status of the developing seed. New Phytologist 182, 17-30.
| Crossref | Google Scholar |
Brodersen KE, Nielsen DA, Ralph PJ, Kühl M (2014) A split flow chamber with artificial sediment to examine the below-ground microenvironment of aquatic macrophytes. Marine Biology 161, 2921-2930.
| Crossref | Google Scholar |
Burnham KP, Anderson DR (2004) Multimodel inference: understanding AIC and BIC in model selection. Sociological Methods & Research 33, 261-304.
| Crossref | Google Scholar |
Chung H, Kim S, Kim K-T, Hwang B-G, Kim H-J, Lee S-J, Lee Y-H (2019) A novel approach to investigate hypoxic microenvironment during rice colonization by Magnaporthe oryzae. Environmental Microbiology 21, 1151-1169.
| Crossref | Google Scholar |
Colmer TD (2003) Long-distance transport of gases in plants: a perspective on internal aeration and radial oxygen loss from roots. Plant, Cell & Environment 26, 17-36.
| Crossref | Google Scholar |
Colmer TD, Winkel A, Pedersen O (2011) A perspective on underwater photosynthesis in submerged terrestrial wetland plants. AoB PLANTS 2011, plr030.
| Crossref | Google Scholar |
Dacey JWH (1980) Internal winds in water lilies: an adaptation for life in anaerobic sediments. Science 210, 1017-1019.
| Crossref | Google Scholar |
Downie JA (2005) Legume haemoglobins: symbiotic nitrogen fixation needs bloody nodules. Current Biology 15, R196-R198.
| Crossref | Google Scholar |
Eklund L (2000) Internal oxygen levels decrease during the growing season and with increasing stem height. Trees (Berlin) 14, 177-180.
| Crossref | Google Scholar |
Erdmann B, Wiedenroth EM (1988) Changes in the root system of wheat seedlings following root anaerobiosis: III. Oxygen concentration in the roots. Annals of Botany 62, 277-286.
| Crossref | Google Scholar |
Geigenberger P, Fernie AR, Gibon Y, Christ M, Stitt M (2000) Metabolic activity decreases as an adaptive response to low internal oxygen in growing potato tubers. Biological Chemistry 381, 723-740.
| Crossref | Google Scholar |
Gibbs J, Greenway H (2003) Mechanisms of anoxia tolerance in plants. I. Growth, survival and anaerobic catabolism. Functional Plant Biology 30, 1-47.
| Crossref | Google Scholar |
Ho QT, Verboven P, Fanta SW, Abera MK, Retta MA, Herremans E, Defraeye T, Nicolaï BM (2014) A multiphase pore scale network model of gas exchange in apple fruit. Food and Bioprocess Technology 7, 482-495.
| Crossref | Google Scholar |
Holdsworth MJ, Gibbs DJ (2020) Comparative biology of oxygen sensing in plants and animals. Current Biology 30, R362-R369.
| Crossref | Google Scholar |
Hunt S, Gaito ST, Layzell DB (1988) Model of gas exchange and diffusion in legume nodules: II. Characterisation of the diffusion barrier and estimation of the concentrations of CO2, H2 and N2 in the infected cells. Planta 173, 128-141.
| Crossref | Google Scholar |
Jiménez JdlC, Pellegrini E, Pedersen O, Nakazono M (2021) Radial oxygen loss from plant roots — methods. Plants 10, 2322.
| Crossref | Google Scholar |
Jordan TE, Whigham DF (1988) The importance of standing dead shoots of the narrow leaved cattail, Typha angustifolia L. Aquatic Botany 29, 319-328.
| Crossref | Google Scholar |
Koch MS, Johnson CR, Madden CJ, Pedersen O (2022a) Irradiance, water column O2, and tide drive internal O2 dynamics and meristem H2S detection in the dominant caribbean-tropical atlantic seagrass, Thalassia testudinum. Estuaries and Coasts 45, 2543-2559.
| Crossref | Google Scholar |
Koch MS, Johnson CR, Madden CJ, Pedersen O (2022b) Low irradiance disrupts the internal O2 dynamics of seagrass (Thalassia testudinum) leading to shoot meristem H2S intrusion. Aquatic Botany 181, 103532.
| Crossref | Google Scholar |
Koren K, Brodersen KE, Jakobsen SL, Kühl M (2015) Optical sensor nanoparticles in artificial sediments – a new tool to visualize O2 dynamics around the rhizome and roots of seagrasses. Environmental Science & Technology 49, 2286-2292.
| Crossref | Google Scholar |
Kozaki A, Takeba G (1996) Photorespiration protects C3 plants from photooxidation. Nature 384, 557-560.
| Crossref | Google Scholar |
Laing HE (1940) The composition of the internal atmosphere of Nuphar advenum and other water plants. American Journal of Botany 27, 861-868.
| Crossref | Google Scholar |
Licausi F, Kosmacz M, Weits DA, Giuntoli B, Giorgi FM, Voesenek LACJ, Perata P, van Dongen JT (2011) Oxygen sensing in plants is mediated by an N-end rule pathway for protein destabilization. Nature 479, 419-422.
| Crossref | Google Scholar |
Masepohl B, Witty JF, Riedel KU, Klipp W, Puehler A (1993) Rhizobium meliloti mutants defective in symbiotic nitrogen fixation affect the oxygen gradient in alfalfa (Medicago sativa) root nodules. Journal of Experimental Botany 44, 419-426.
| Crossref | Google Scholar |
Millar AH, Whelan J, Soole KL, Day DA (2011) Organization and regulation of mitochondrial respiration in plants. Annual Review of Plant Biology 62, 79-104.
| Crossref | Google Scholar |
Mincke J, Courtyn J, Vanhove C, Vandenberghe S, Steppe K (2021) Guide to plant-PET imaging using 11CO2. Frontiers in Plant Science 12, 602550.
| Crossref | Google Scholar |
Mommer L, Pedersen O, Visser EJW (2004) Acclimation of a terrestrial plant to submergence facilitates gas exchange under water. Plant, Cell & Environment 27, 1281-1287.
| Crossref | Google Scholar |
Mustroph A, Lee SC, Oosumi T, Zanetti ME, Yang H, Ma K, Yaghoubi-Masihi A, Fukao T, Bailey-Serres J (2010) Cross-kingdom comparison of transcriptomic adjustments to low-oxygen stress highlights conserved and plant-specific responses. Plant Physiology 152, 1484-1500.
| Crossref | Google Scholar |
Møller CL, Sand-Jensen K (2011) High sensitivity of Lobelia dortmanna to sediment oxygen depletion following organic enrichment. New Phytologist 190, 320-331.
| Crossref | Google Scholar |
Nery AA, Calbo AG (1994) Adapting constant-volume manometry for studying gas exchange by bulky plant organs. Journal of the American Society for Horticultural Science 119, 1222-1229.
| Crossref | Google Scholar |
Pedersen O, Vos H, Colmer TD (2006) Oxygen dynamics during submergence in the halophytic stem succulent Halosarcia pergranulata. Plant, Cell & Environment 29, 1388-1399.
| Crossref | Google Scholar |
Pedersen O, Colmer TD, Borum J, Zavala-Perez A, Kendrick GA (2016) Heat stress of two tropical seagrass species during low tides – impact on underwater net photosynthesis, dark respiration and diel in situ internal aeration. New Phytologist 210, 1207-1218.
| Crossref | Google Scholar |
Pedersen O, Revsbech NP, Shabala S (2020) Microsensors in plant biology: in vivo visualization of inorganic analytes with high spatial and/or temporal resolution. Journal of Experimental Botany 71, 3941-3954.
| Crossref | Google Scholar |
Pedersen O, Nakayama Y, Yasue H, Kurokawa Y, Takahashi H, Heidi Floytrup A, Omori F, Mano Y, David Colmer T, Nakazono M (2021) Lateral roots, in addition to adventitious roots, form a barrier to radial oxygen loss in Zea nicaraguensis and a chromosome segment introgression line in maize. New Phytologist 229, 94-105.
| Crossref | Google Scholar |
Pellegrini E, Konnerup D, Winkel A, Casolo V, Pedersen O (2017) Contrasting oxygen dynamics in Limonium narbonense and Sarcocornia fruticosa during partial and complete submergence. Functional Plant Biology 44, 867-876.
| Crossref | Google Scholar |
Peralta Ogorek LL, Pellegrini E, Pedersen O (2021) Novel functions of the root barrier to radial oxygen loss – radial diffusion resistance to H2 and water vapour. New Phytologist 231, 1365-1376.
| Crossref | Google Scholar |
R Core Team (2022) R: A language and environment for statistical computing. (R Foundation for Statistical Computing: Vienna, Austria). Available at https://www.R-project.org/
Revsbech NP (1989) An oxygen microsensor with a guard cathode. Limnology and Oceanography 34, 474-478.
| Crossref | Google Scholar |
Rich SM, Ludwig M, Pedersen O, Colmer TD (2011) Aquatic adventitious roots of the wetland plant Meionectes brownii can photosynthesize: implications for root function during flooding. New Phytologist 190, 311-319.
| Crossref | Google Scholar |
Rich SM, Pedersen O, Ludwig M, Colmer TD (2013) Shoot atmospheric contact is of little importance to aeration of deeper portions of the wetland plant Meionectes brownii; submerged organs mainly acquire O2 from the water column or produce it endogenously in underwater photosynthesis. Plant, Cell & Environment 36, 213-223.
| Crossref | Google Scholar |
Romanov VI, Gordon AJ, Minchin FR, Witty JF, Skøt L, James CL, Borisov AY, Tikhonovich IA (1995) Anatomy, physiology and biochemistry of root nodules of Sprint-2 Fix-, a symbiotically defective mutant of pea (Pisum sativum L.). Journal of Experimental Botany 46, 1809-1816.
| Crossref | Google Scholar |
Rudolph N, Esser HG, Carminati A, Moradi AB, Hilger A, Kardjilov N, Nagl S, Oswald SE (2012) Dynamic oxygen mapping in the root zone by fluorescence dye imaging combined with neutron radiography. Journal of Soils and Sediments 12, 63-74.
| Crossref | Google Scholar |
Sand-Jensen KAJ, Pedersen O, Binzer T, Borum J (2005) Contrasting oxygen dynamics in the freshwater isoetid Lobelia dortmanna and the marine seagrass Zostera marina. Annals of Botany (London) 96, 613-623.
| Crossref | Google Scholar |
Schmidt RR, Weits DA, Feulner CFJ, van Dongen JT (2018) Oxygen sensing and integrative stress signaling in plants. Plant Physiology 176, 1131-1142.
| Crossref | Google Scholar |
Schmälzlin E, van Dongen JT, Klimant I, Marmodée B, Steup M, Fisahn J, Geigenberger P, Löhmannsröben H-G (2005) An optical multifrequency phase-modulation method using microbeads for measuring intracellular oxygen concentrations in plants. Biophysical Journal 89, 1339-1345.
| Crossref | Google Scholar |
Scholander PF (1947) Analyzer for accurate estimation of respiratory gases in one-half cubic centimeter samples. Journal of Biological Chemistry 167, 235-250.
| Crossref | Google Scholar |
Setter TL, Kupkanchanakul T, Kupkanchanakul K, Bhekasut P, Wiengweera A, Greenway H (1987) Concentrations of CO2 and O2 in floodwater and in internodal lacunae of floating rice growing at 1–2 metre water depths. Plant, Cell & Environment 10, 767-776.
| Google Scholar |
Shaw DS, Honeychurch KC (2022) Nanosensor applications in plant science. Biosensors 12, 675.
| Crossref | Google Scholar |
Sorrell BK, Tanner CC (2000) Convective gas flow and internal aeration in Eleocharis sphacelata in relation to water depth. Journal of Ecology 88, 778-789.
| Crossref | Google Scholar |
Sorz J, Hietz P (2008) Is oxygen involved in beech (Fagus sylvatica) red heartwood formation? Trees 22, 175-185.
| Crossref | Google Scholar |
Spalding MH, Stumpf DK, Ku MSB, Burris RH, Edwards GE (1979) Crassulacean acid metabolism and diurnal variations of internal CO2 and O2 concentrations in Sedum praealtum DC. Functional Plant Biology 6, 557-567.
| Crossref | Google Scholar |
Stevens KJ, Peterson RL, Reader RJ (2002) The aerenchymatous phellem of Lythrum salicaria (L.): a pathway for gas transport and its role in flood tolerance. Annals of Botany (London) 89, 621-625.
| Crossref | Google Scholar |
Stiles W (1960) The composition of the atmosphere (oxygen content of air, soil, intercellular spaces, diffusion, carbon dioxide and oxygen tension). In ‘Encyclopedia of plant physiology, plant respiration inclusive fermentations and acid metabolism. Vol. XII (Part 2)’. (Ed. W Ruhland) pp. 114–148. (Springer Verlag: Heidelberg, Germany)
Symonds MRE, Moussalli A (2011) A brief guide to model selection, multimodel inference and model averaging in behavioural ecology using Akaike’s information criterion. Behavioral Ecology and Sociobiology 65, 13-21.
| Crossref | Google Scholar |
Thoday D (1913) On the capillary eudiometric apparatus of Bonnier and Mangin for the analysis of air in investigating the gaseous exchanges of plants. Annals of Botany os-27, 565-573.
| Crossref | Google Scholar |
Tjepkema JD, Cartica RJ (1982) Diffusion limitation of oxygen uptake and nitrogenase activity in the root nodules of Parasponia rigida Merr. and Perry. Plant Physiology 69, 728-733.
| Crossref | Google Scholar |
Van Breusegem F, Bailey-Serres J, Mittler R (2008) Unraveling the tapestry of networks involving reactive oxygen species in plants. Plant Physiology 147, 978-984.
| Crossref | Google Scholar |
Voesenek LACJ, Bailey-Serres J (2015) Flood adaptive traits and processes: an overview. New Phytologist 206, 57-73.
| Crossref | Google Scholar |
Voesenek LACJ, Colmer TD, Pierik R, Millenaar FF, Peeters AJM (2006) How plants cope with complete submergence. New Phytologist 170, 213-226.
| Crossref | Google Scholar |
Weits DA, Kunkowska AB, Kamps NCW, Portz KMS, Packbier NK, Nemec Venza Z, Gaillochet C, Lohmann JU, Pedersen O, van Dongen JT, Licausi F (2019) An apical hypoxic niche sets the pace of shoot meristem activity. Nature 569, 714-717.
| Crossref | Google Scholar |
Weits DA, van Dongen JT, Licausi F (2021) Molecular oxygen as a signaling component in plant development. New Phytologist 229, 24-35.
| Crossref | Google Scholar |
Wiengweera A, Greenway H, Thomson CJ (1997) The use of agar nutrient solution to simulate lack of convection in waterlogged soils. Annals of Botany 80, 115-123.
| Crossref | Google Scholar |
Footnotes
1 Below detection limits which is approximately 0.007 kPa for O2 microelectrodes (Revsbech 1989).